All published articles of this journal are available on ScienceDirect.
Sheep Hip Arthroplasty Model of Failed Implant Osseointegration
Abstract
Early secure stability of an implant is important for long-term survival. We examined whether micromotion of implants consistently would induce bone resorption and formation of a fibrous membrane and thereby prevent osseointegration.
One micromotion implant was inserted into one of the medial femoral condyles in ten sheep. The micromotion device consists of an anchor bearing a PMMA implant and a PE plug. During each gait cycle the PE plug will make the PMMA implant axially piston 0.5 mm. After 12 weeks of observation the bone specimens were harvested and a post-mortem control implant was inserted into the contra-lateral medial femoral condyle.
Histomorphometrical evaluation showed that the surface on the implant observed for 12 weeks was covered by fibrous tissue. The control implants were covered by lamellar bone. No difference was found with respect to the volume fraction of lamellar bone in a 1 mm zone around the implants.
This study indicates that implant micromotion is sufficient to induce bone resorption and formation of a fibrous membrane.
INTRODUCTION
Long-term survival of total joint replacements relies upon initial mechanical stability in order to prevent migration and prosthetic loosening. Radiostereometrical analysis has shown that early subsidence is a strong predictor for later revision [1, 2]. Therapies that can secure initial mechanical stability and prevent early implant migration could potentially be able to increase long-term implant survival.
A prerequisite for early implant migration to take place is loss of implant osseointegration and mechanical stability, resorption of peri-prosthetic bone and formation of a fibrous membrane [3-6]. Wear particles are not obligate for early bone resorption and implant migration. This is supported by studies that have shown that bone resorption can be initiated by implant movement or pressure alone without the presence of wear particles [7, 9]. Late implant loosening is usualy associated with excessive wear particles [5]. Several studies suggest that the cascade of late implant loosening is initiated by wear debris generated at the joint articulation and subsequently transported through the “effective joint space” into the peri-prosthetic bone where macrophages and osteoclasts are activated [5, 10]. We have previously shown that hydroxy-apatite coated implants has a sealing effect against migration of wear debris particles [11]. Fibrous tissue at the bone-implant interface generated by early implant micromotion might predispose transport of wear-particles from the joint articulation to the bone-implant interface. We have previously shown that polyethylene particles aggravate the formation of a fibrous membrane around implants subjected to micromotion [12].
It could be that the first step in loss of implant osseointegration is micromotion-induced bone resorption and formation of a fibrous membrane. Implants not proper fixated initially would begin to conduct micro movements and thereby induce bone resorption. The aim of this study was to establish a model of early failed implant osseointegration. We wanted to investigate the effect of controlled micromotion on implants inserted intra-articular with access to synovial fluid and subjected to 12 weeks.
We tested the hypothesis that implant-micromotion for 12 weeks would prevent osseointegration, increase resorption of peri-implant bone and induce formation of fibrous tissue in a sheep model.
MATERIALS AND METHODS
Study Design
We used 10 skeletally mature sheep with a mean weight of 39 kg (range, 35-40 kg). Each sheep had one loaded micromotion device (Fig. 1) inserted into one of the medial femoral condyles. After a 12 weeks observation period the animals were euthanized and both medial femoral condyles were removed and a similar micromotion device was inserted into the contralateral medial femoral condyle. The micromotion device inserted post-mortem acts as a time zero control implant. We alternated the intervention side with each animal.
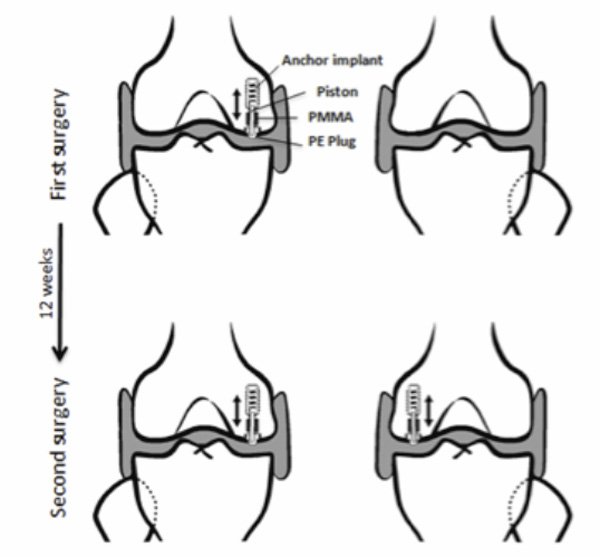
Schematic drawing showing implant position in the medial femoral condyle.
Our Institutional Animal Care and Use Committee approved the study. Institutional guidelines for the treatment and care of experimental animals were followed.
Implants
We used 20 custom-made micromotion implant devices. Our micromotion device consists of an anchor that contains a spring-loaded piston. A cylindrical polymethylmethacrylate (PMMA) implants was screwed onto the distal part of the piston (Fig. 1). A polyethylene (PE) plug was screwed onto the distal part of the piston superficial to PMMA implants. The PMMA was molded and had a height of 10.0 mm and a diameter of 7.5 mm. The micromotion device allowed controlled axial implant movement of the 7.5 mm PMMA in a cavity with a diameter of 7.5 mm. The PMMA implant pistons 0.5 mm when loaded during each gait cycle. All implants were sterilized by gamma irradiation (25-50 kGy for 16 hours, Codan Steritex, Espergaerde, Denmark).
Surgery
All surgery was done using sterile conditions and with the sheep under general anesthesia. The animals were given 1 g of Rocephalin (ceftriaxone) preoperatively. At primary surgery a micromotion device was inserted into one of the medial femoral condyles. We exposed the knee through an anteromedial approach. Maximal flexion of the knee presented the weight-bearing area of the medial femoral condyle. A k-wire was inserted perpendicular to the surface of the central portion of the condyle. We used a steep drill to create a distal cavity (6.0 mm diameter by 10.0 mm long) and a superficial cavity (7.5 mm diameter by 20.0 mm long). We drilled with two rotations per second and continuously irrigated the drill in order to prevent thermal bone trauma.
After drilling, we irrigated the bone cavity in order to remove bone debris that potentially could get stock in the bone-implant interface and prevent the controlled implant micromotion. Next, we inserted the anchor implant into the 6.0 mm deep part of the cavity. Onto the anchor we screwed the PMMA implant and PE plug. Our PMMA implants were inserted into exact-fit and special attention was made to ensure that all inserted micromotion devices would be able to axially move when loaded and return to initial position when unloaded. The PE plug protrusion into the joint was at least 0.5 mm in order to secure full displacement of PMMA implant when loaded. No PE particles were added. Soft tissue was closed in layers and full range of motion of the knee was confirmed. All sheep were weight bearing within 5 days surgery.
After 12 weeks of observation all sheep were euthanized, and the distal femur with an implant was collected for preparation and analysis. At specimen harvest, proper micromotion function of all implants was confirmed. Post- mortem, we inserted a micromotion device into the contralateral knee. Insertion of the micromotion device was done using a similar procedure as used during first surgery. Immediately after second surgery the contralateral knee was collected and prepared for analysis.
Specimen Preparation
All bone specimens were instantly stored at -20°C. We prepared one specimen from each medial femoral condyle. Each specimen containing the PMMA implant was cut perpendicular to the long axis of the implant using a water- cooled band saw (Exact Apparatebau, Nordenstedt, Germany) (Fig. 2). The specimen with a thickness of 8 mm was fixed in 70 % ethanol and used for later histological and histomorphometrical analysis. Preparation and subsequent evaluation were blinded.
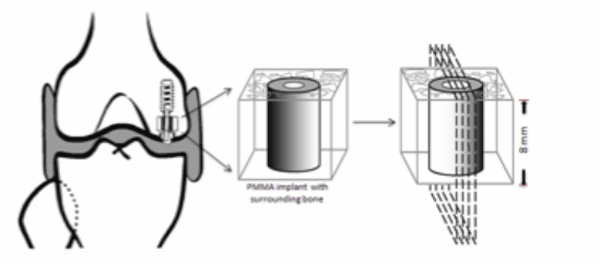
Schematic diagram showing the specimen preparation. Each bone-implant specimen is embedded and cut into four slides for histomorphometrical analysis.
Histology and Histomorphometry
Each bone-implant specimen was gradually dehydrated in ethanol (70%-96%), and infiltrated and embedded in Technovit 7100 (FIRMA). Four vertical uniform random sections were cut with a hard tissue microtome (KDG-95, MeProTech, Heerhugowaard, The Netherlands) around the center part of each implant as described by Overgaard et at [13]. Before making the sections, the implant was randomly rotated around its long axis. The sections were cut parallel to this axis. These techniques provide reliable results with negligible bias [14]. The 25-µm thick sections were cut with a distance of 400 µm, and surface stained with 0.1 % toluidine blue (pH 7) (Sigma-Aldrich, St. Louis, Missouri) and mounted on glass.
Blinded histomorphometrical analysis was done using a stereological software program (newCAST, Visiopharm A/S, Horsholm, Denmark). Fields of vision from a light microscope were transferred to a computer monitor. Region of interest was defined as the area from the implant surface extending 1.0 mm into the circumferential peri-implant zone. Surface fractions of bone-to-implant contact were estimated using sine-weighted lines [15], whereas volume fractions of bone density were estimated by point counting [16] in a 1000 µm peri-implant zone.
Discrimination between woven and lamellar bone was done based on morphological characteristics: woven bone had random orientation of osteocytes, large osteocytes, and random orientation of collagen fibres whereas lamellar bone was arranged in parallel lamellae. Fibrous tissue appeared as well-organized bundles of fibres with spindle-shaped cells. Bone marrow appeared as a disorganized cell-rich structure with empty areas representing dissolved fat.
Statistical Analysis
We used Intercooled Stata 9.0 (Stata Inc., College Station, TX, USA) for statistical analysis. Statistical analyses were done on ratios between paired data, which were not normally distributed. All variables were therefore log- transformed and Student’s paired t-test was performed on absolute differences between normally distributed log- transformed paired data. Two tailed p-values below 0.05 were considered statistically significant. Results are presented as medians of relative differences between the paired data. The 95% confidence intervals were obtained by back transformation of log-transformed data unless otherwise stated.
RESULTS
Surgery
All sheep completed the 12-weeks observation period. No clinical sign of infection were present at time of euthanization.
Histology
The most striking difference between the two treatment groups was the presence of a fibrous membrane around the micromotion implants (Fig. 3). The thickness of the membrane was approximately 500 µm. The fibrous membrane consisted of two layers. One layer close to the implant surface with a thickness of approximately 100 µm and another layer away from the implant with a thickness of approximately 400 µm. The layer closest to the implant surface consisted of dense parallel fibers and few spindle- shaped cells. The layer away from the implant surface consisted of less dense disorganized fibers with irregular formed cells. Furthermore, this layer contained areas with disorganized cartilage in which endochondral ossification were seen (Fig. 4).
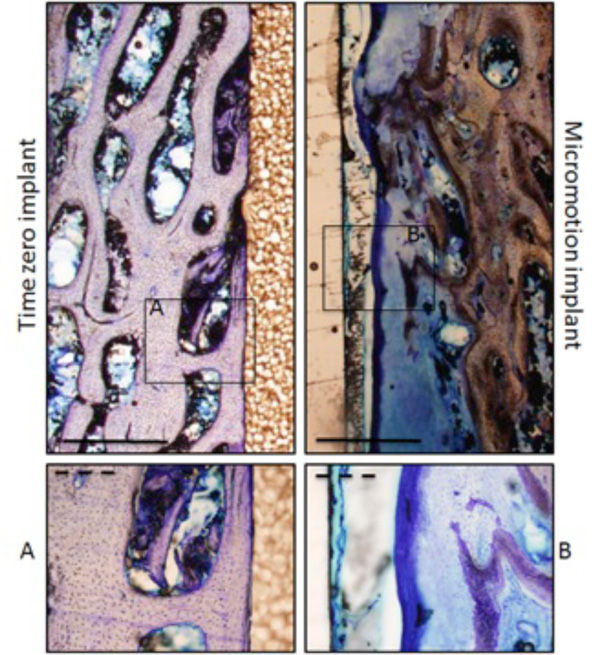
Representative photomicrographs of samples from the same animal. The samples were stained with 0.1 % toluidine blue. Note the surface contact of lamellar bone on the time zero implant and the fibrous tissue around the micromotion implant. Solid bar = 1 mm. Dotted bar = 0.3 mm.
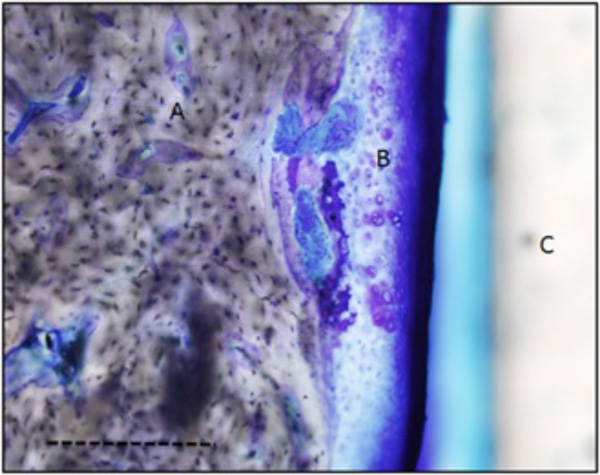
Photomicrograph showing cartilage tissue in one of the fibrous membranes surrounding a micromotion implant. The sample was stained with 0.1 % toluidine blue. A = lamellar bone. B= cartilage. C = implant. Dotted bar = 0.3 mm.
The main histological difference between the two treatment groups with respect to bone was the presence of small bony chips around the time zero implants. The bony chips were characterized as small fragment of lamellar bone sharp edges. These bony chips represent remnants of bone fragment created by drilling the cavity. No bony chips were seen in the micromotion group.
Histomorphometry
The surfaces from the micromotion implants were covered by 93 % (95% CI: 84 % - 99 %) of fibrous tissue compared to 0% (95% CI: 0 % - 0%) in the time zero implant group (p < 0.0001). The surface of the time zero implants were instead covered by 91 % (95% CI: 85 % - 97 %) of lamellar bone compared to only 4 % (95% CI: 0 % - 13 %) for the micromotion implants (p < 0.0001) (Fig. 5). No woven bone in contact with the implant surfaces were detected in any of the two groups.
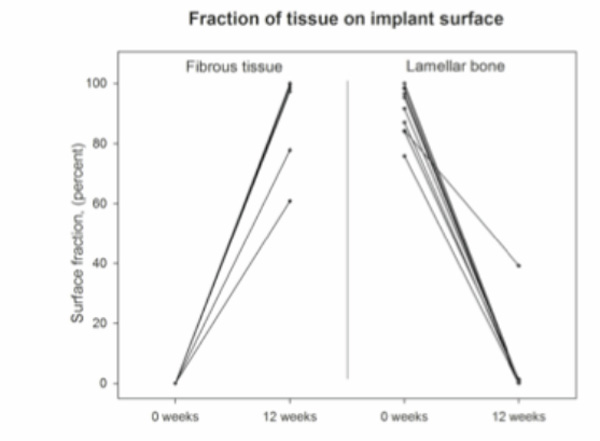
Fraction of tissue on implant surface. Paired data connected by line.
Twelve weeks of micromotion resulted in 24 % (95% CI: % - 35%) fibrous tissue in a 1 mm zone around the micromotion implants compared to 0 % (95% CI: 0% - 0%) around the time zero implants without micromotion (p = 0.0007). Seven percent (95% CI: 2% - 11%) of woven bone was detected around the micromotion implants compared to 0 % around the time zero implants (95% CI: 0% - 0%) (p = 0.007). We found no significant difference in the volume- fraction of lamellar bone between the two groups (p = 0.19) (Fig. 6).
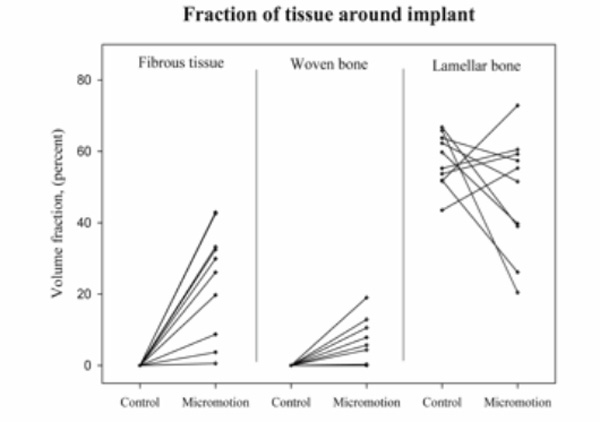
Tissue-volume fractions in a 0-1 mm zone around implants. Paired data connected by line.
DISCUSSION
The purpose of this study was to investigate the effect of 12- weeks controlled micromotion on implant osseointegration. We wanted to investigate whether micromotion for 12 weeks would prevent osseointegration and instead induce bone resorption and formation of a fibrous membrane. We found that 12 weeks of micromotion induced resorption of lamellar bone in contact with the implants surface and formation of a fibrous membrane.
Our experimental model was designed to represent the portion of a cemented human hip replacement placed into cancellous bone. Early migration of clinical hip replacements is associated with a high risk an early failure [1]. Our experimental model allows controlled implant micromotion and is designed to imitate a clinical joint replacement subjected to early migration. We placed our implants intra-articular in the medial femoral condyle in order to imitate the clinical situation were implants are direct load-bearing and synovial fluid has access to the bone-implant interface. Ovine cancellous bone was chosen since in closely resembles human cancellous bone [17]. The paired design allowed us to eliminate the biological difference between individuals.
The aim of the study was to establish a model of failed early implant osseointegration. We wanted to investigate whether 12 weeks of implant micromotion would prevent osseointegration, induce bone resorption and induce formation of fibrous tissue. In order to investigate the time course of bone resorption and to demonstrate that all implant initially were in exact-fit bone contact we choose our control implant to be inserted post- mortem. Our post-mortem implants were intended to represent time zero of implant insertion in a paired design. As a consequence of inserting of control implant post-mortem both presence of micromotion and 12 weeks observation exists between the two treatment groups. However, we known from previous studies, using a loaded intra-articular porous coated Ti- implant model, that stable conditions does not induce bone resorption but allows formation of new bone around implants inserted in both gap-fit and press-fit [12, 18]. Any difference between the two treatment groups in this study is therefore attributed to the effect of micromotion.
In this study, controlled micromotion and 12 weeks observation was sufficient in order to convert lamellar bone in contact with implant surface into a fibrous membrane. Likely explanation for the fibrous membrane formation is that micromotion alone is able to activate pro-inflammatory cells and induce bone resorption followed by fibrous membrane formation. Our finding is supported by other experimental studies in which instability leads to bone resorption [19, 20]. These studies support our finding, since wear-particles are not present at this early stage.
The effects of micromotion in this study were strongest at the implant surface and diminished with increasing distance from the implant surface. At the surface, we observed a dense fibrous membrane. Further away from the surface, we observed more disorganized fibrous tissue. Outermost from the surface, lamellar bone was present. No difference in the density of this lamellar bone was observed between the two treatment groups. We know from other studies that movement affects bone formation in a dose-response manner [20]. The lowest magnitude of movement that will not induce osteolysis is still unknown.
It is well known from in vivo and in vitro studies that wear- debris alone can induce osteolysis [21, 22]. With this study, we show that 12 weeks of micromotion is sufficient to induce bone resorption. We know from previous studies that particulate polyethylene can aggravate the effect of movement and induce a stronger bone resorptive response [12]. It could be that aseptic osteolytic loosening of total hip replacements is a temporal and spatial process. Temporal since aseptic looseling occurs in different stages. One stage with early migration where micromotion might induce formation of a fibrous membrane and thereby enlarge the effective joint space. A second stage where wear-debris present at the implant-tissue interfaces aggravates the osteolysis and leads to implant failure. Spatial since wear-debris is generated at the joint articulation, but needs to be transported to the implant-tissue interface. The presence of a fibrous membrane aids in this transport while strong osseointegration exerts a “sealing effect” and protects against transport [11].
Our findings suggest that strategies improving early implant fixation may be beneficial for long-term implant survival. Strategies should be directed at improving early osseointegration and thereby creating a stable implant fixation since micromotion could be the first step in osteolytic cascade. The result of this study allows us to use our micromotion model for further investigation in treatments that potentially can reduce peri-implant osteolysis.
CONCLUSION
In conclusion, this study suggests that micromotion is sufficient to prevent implant osseointegration and to induce bone resorption and formation of a fibrous membrane. Furthermore, this study demonstrates a model were interventions against bone resorption can be investigated. However, the results should be extrapolated with caution since only one magnitude of micromotion and one observation period was investigated. Studies investigating cellular mechanism behind micromotion induced bone resorption and the dose- response relationship between micromotion and bone resorption are needed.
CONFLICT OF INTEREST
The authors confirm that this article content has no conflict of interest.
ACKOWLEDGEMENTS
This study was supported by The Danish Ministry of Higher Education and Science. The authors wish to thank Jane Pauli, Orthopaedic research Laboratory, Aarhus University Hospital, for technical expertise.