All published articles of this journal are available on ScienceDirect.
An Ultrasound Assisted Anchoring Technique (BoneWelding® Technology) for Fixation of Implants to Bone – A Histological Pilot Study in Sheep
Abstract
The BoneWelding® Technology offers new opportunities to anchor implants within bone. The technology melted the surface of biodegradable polymer pins by means of ultrasound energy to mould material into the structures of the predrilled bone. Temperature changes were measured at the sites of implantation in an in vitro experiment. In the in vivo part of the study two types of implants were implanted in the limb of sheep to investigate the biocompatibility of the method. One implant type was made of PL-DL-lactide (PLA), the second one was a titanium core partially covered with PLA. Healing period was 2 and 6 months, with 3 sheep per group. Bone samples were evaluated radiologically, histologically and histomorphometrically for bone remodeling and inflammatory reactions. Results demonstrated mild and short temperature increase during insertion. New bone formed at the implant without evidence of inflammatory reaction. The amount of adjacent bone was increased compared to normal cancellous bone. It was concluded that the BoneWelding® Technology proved to be a biocompatible technology to anchor biodegradable as well as titanium-PLA implants in bone.
INTRODUCTION
The anchorage of implants in bone is an integral part of modern fixation techniques in orthopedics. Metal implants usually fulfill all requirements in healthy and normal bone. However, if bone structure is weak, the holding power may not be sufficient for a stable fixation [1, 2]. In osteoporotic bone mechanical stability is a great challenge with a decrease in bone mass and increased brittleness [3]. Methods for immediate and overall improved primary stability are required to meet these challenges emerging with an increasingly aging population.
The ultrasound assisted anchoring technology (BoneWelding® technology) has been developed to address this problem. It combines the liquefaction and resorbability of a polymer to achieve better stability compared to screws alone. The ultrasonic vibrations cause liquefaction of the polymer in contact with the bone (Fig. 1). The liquid phase infiltrates the cancellous cavities of the adjacent bone and adheres to the structures. Re-solidification initiates as soon as the supply of ultrasonic energy is stopped.
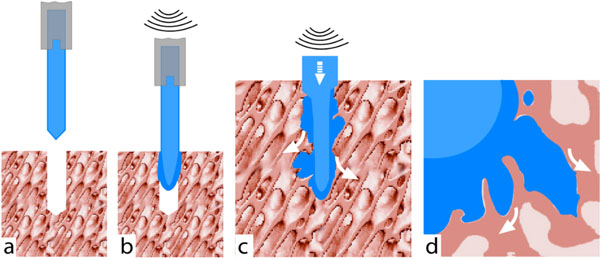
An active ultrasonic sonotrode drives the polymer pin into the bone (a). Heat generating shearing forces at the contact face (b) liquefy polymer, which penetrates into the bone spaces (c, d).
Biomechanical tests in healthy and osteoporotic human cadaver and synthetic bones already demonstrated improved mechanical anchorage of the polymeric implants that were inserted using the BoneWelding® technique compared to conventional metal screws [4, 5].
This study included an in vitro part for temperature measurements on cadaver bone and an in vivo part in sheep to evaluate the biocompatibility of the technology after medium term implantation periods. The in vitro insertions were undertaken to quantify the thermal impact and compare it to heat exposure during drilling [6, 7]. The same setup was used in the in vivo part to test for signs of potential damage to the adjacent bone due to heat generated by insertion.
The primary focus of the present study was the in vivo-biocompatibility of the ultrasonic insertion by means of qualitative histological assessment of bone response. It was assumed that the excellent biocompatibility of the implant components, PLA [8, 9] and titanium [10, 11], would not be altered by the insertion technique. Possible changes in tissue reaction could then be attributed to the ultrasound induced liquefaction process alone. A poly-L-DL-Lactide was used as polymeric cylindrical dowel implant in both parts and in the in vivo part also a partial PLA coated titanium implant (Ti-PLA) was investigated. The Ti-PLA pin had a similar, dowel like form as the polymer pin and the coating was of the same PLA material. The diameter was smaller, which lead to a gap between the drilled bone cavity and the titanium surface. This design facilitated anchoring only by the polymer part and avoided direct transmission of ultrasonic energy from metal to bone as an unknown factor in the setup. The performance of implants was tested in cancellous and cortical bone to establish results for several clinical applications.
MATERIALS AND METHODOLOGY
Implants
The PLA pin consisted of a co-polymer of 70% L-lactide and 30% DL-lactide (Poly-L-DL-Lactide, Resomer® LR 708, Boehringer Ingelheim, Germany) which was molded into a cylindrical dowel of 3.5 mm diameter with a tapered tip and 25 mm length (Fig. 2). The titanium-PLA implants had a length of 10 mm and a diameter of 2.8 mm. The implant was machined out of grade 4 titanium and surface treated by gridblasting and subsequent acid etching. Polymer was layered at the surface in small stripes (1 mm in width, 5 mm in length, and 1 mm in height) at 180° opposite of each other through a thermal sintering process. The remaining 5-mm titanium surface of the Ti-PLA implant was left uncovered. At the proximal end a thread was introduced at the inside, where an adapter piece could be introduced to serve as a coupling device to the sonotrode tip of the ultrasound equipment. After insertion and removal of the ultrasound device the adapter was replaced by a small screw.
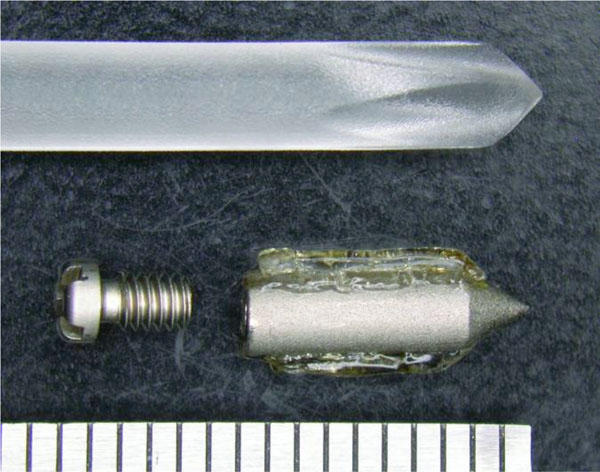
Pictures of the implants. The pure PLA-pin (top) was fixed directly at the sonotrode, while an adaptor was used for the titanium-PLA pin (Ti-PLA)(bottom, above the millimeter scale). Polymer stripes were sintered at the titanium core.
The diameter of the implants was calculated such, that enough material was available to infiltrate the surrounding bone. The implants were sterilized using gamma irradiation (60Co-radiation, 25kGy).
Ultrasound Device
A commercially available ultrasound device was used (Branson E-150, Branson Ultrasonics SA, Carouge, Switzerland; 20kHz, amplitude of max. 60 µm = 150W, set at 50%). The device was covered with a sterile plastic cover (rolled arthroscopy camera drape, mtp GmbH, Tuttlingen, Germany). The metallic implant holder of the device was disinfected using alcohol and chlorhexidine (Hibiscrub, SSL Healthcare, Switzerland).
In Vitro Temperature Measurements
An in vitro study was conducted to document the thermal conditions of implantation in the osseous tissue close to the implant-bone interface. The same setup was used as in the in vivo part, but with fresh thawed sheep tibiae and femora. Bones where kept at 37°C, clamped fully submerged in a water basin. In total 3 femora and 4 tibiae were investigated, leading to 7 to 8 insertions for each area. Four equally spaced 0.5 mm holes were drilled parallel to the implantation hole at a distance of 3 mm from center point to center point. The distance between the initial pin surface and thermocouple (Sheathed thermocouple, J Type, ø 0.5 mm, MDW, Switzerland) was therefore about 1 mm. The depth of the thermocouple holes was 3 mm. Reference temperature was measured in a distance of 2 cm from the pin hole. Data were collected 17 to 18 times per second (Agilent 34970A Data Acquisition Switch Unit, Santa Clara, CA, USA) for app. 60 seconds, starting 10 seconds before insertion. Measurements were visualized by box plots.
Animal Model
Six adult, female Swiss Alpine sheep between 2-4 years of age and with an average weight of 71 kg (range 66-84 kg) served as well established animal model for human bone healing [12-15]. Animal experiment was conducted according to the Swiss Law of animal protection and welfare and was permitted through the official, Swiss authorities (permission # 92/2002). Perioperative care, inhalation anesthesia and postoperative analgesia and antibiosis was performed according to standard protocols as described elsewhere [13].
Animals were sacrificed after 2 and 6 months period with 3 animals per time point. A total of 48 implants were placed in the distal femur and proximal tibia. The target areas (Fig. 3) were chosen such that 8 implants per sheep, 4 of each type, were placed in cancellous and cortical bone to test in various bone types. Each area contained one PLA and one Ti-PLA implant. Minimum distance between all implants was 10 mm. Implant cavities were prepared by drilling (3 mm in diameter, 10 mm depth) and additional tapping in cortical areas (tap for 3.5 mm cortex screws, Synthes®, Oberdorf, Switzerland) to create open space for the liquefied polymer. All implants were inserted to a depth of 10 mm while applying ultrasonic energy for about 1 sec. Thereafter, the implant was left to allow re-solidification for about 10 seconds, before the tip of the sonotrode was disconnected. The protruding part of the PLA pin was cut off with a wire cutter as close to the bone surface as possible. The screw cap of the Ti-PLA implant was inserted using a special screwdriver.
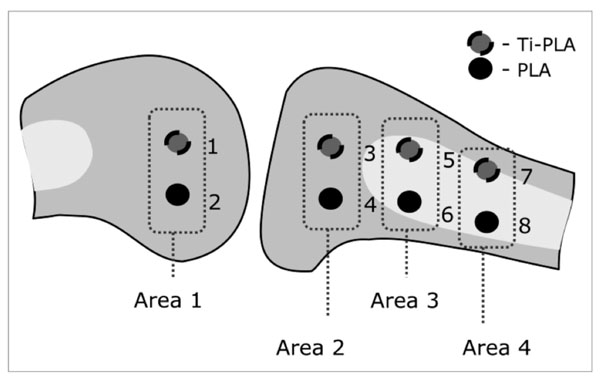
Schematic drawing of the implantation sites (1-8) in cancellous bone of the femur (area 1) and cancellous and cortical bone in the proximal tibia (area 2-4). One implant of PLA and Ti-PLA type was inserted in each area.
Macroscopic and Radiographic Evaluation
After sacrifice the implant sites were examined for signs of inflammation. Radiographic follow-up was performed immediately after surgery and after sacrifice of the animals. Contact radiographs (Faxitron X-Rays systems, Hewlett Packard, Oregon, USA; set at 45 kV, 1 sec) were taken in two levels of the complete bones and the singled implantation areas. Each pair of implants was cut out in blocks and processed for undecalcified bone histology as described elsewhere [13]. The proximal portion of the implant including the cortex was cut lengthwise, whereas the distal portion including the cancellous bone or bone marrow was cut transversely to the implant axis exposing the full diameter of the implant. One ground section (150 µm) and two thin sections (6 µm) were surface stained with toluidine blue and von Kossa / McNeal method. Contact microradiographs were taken from the ground sections. Thin sections of the Ti-PLA implants were prepared as statuary example of 12 samples (6 samples per observation period).
Qualitative evaluation of the histological sections was carried out microscopically (DMR, Leica, Switzerland) placing emphasis on bone resorption, new bone and fibrous tissue formation close to the implant. In addition the interface between the implant and bone was assessed carefully. Cellular aspects were analyzed in thin sections, bone morphology in ground sections.
Quantitative evaluation was performed to compare changes in new bone formation adjacent to the implant observed in the qualitative evaluation in cancellous bone samples. Digital images (macroscope M 420 and digital camera DC 200, Leica, Switzerland) of ground sections that were cut longitudinally to the axis of the implant served as data source. The bone area surrounding the implant was divided in three similar sized and predefined sectors (Fig. 4) to calculate changes of bone at the implant in comparison to the bone in the periphery. Sector 1 was adjacent to the implant, sector 2 in the middle and sector three was in the periphery of the section. The extent of sector 1 was defined to cover the remodeling and new bone formation at the implant, whereas sector 2 and 3 were not influenced by remodeling and served as reference. The cancellous bone structures in the sectors were identified and colored manually (Adobe® Photoshop® 7.0, Adobe Systems, San Jose, CA) and all other structures were covered with a black mask to reduce false detection in image analysis software (Qwin® v.1, Leica Microsystems, Cambridge, UK). Bone matrix of each side of the implant was measured separately. Bone area was calculated from the measured bone area in relation to the total area of the corresponding sector.
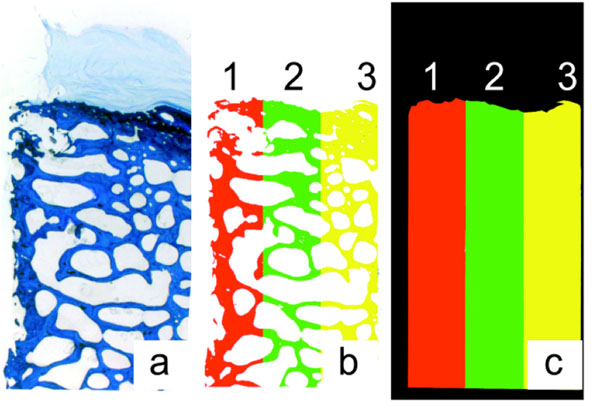
Histomorphometrical bone area measurement. Bone matrix (a) was split into 3 sectors (b), sector 1 was directly at the implant. The percentage of bone in each sector was calculated with the bone area (b) and the total area (c).
Statistical analysis was performed to compare bone area between sectors and determine relevance of implant type, observation period and implantation areas. Analysis was performed using STATA (version 10.0, Statacorp, Texas, USA), using a general linear mode and mixed-effects restricted maximum likelihood (REML) regression. P-values <0.05 were considered to be statistically significant.
Semi-quantitative evaluation was done in thin sections. Full sections were scanned for presence of osteoclasts, giant foreign body cells and lymphocytes. Cellular events were counted in regard to a reaction to the implant.
RESULTS
In Vitro Temperature Measurements
The time dependency was characterized by a rather sharp initial peak for 10 seconds that drops back to ambient temperature in 50 to 60 seconds (Fig. 5). Small temperature differences among the thermocouples could be recorded. Maximal temperature increases between 4 to 11°C were measured with medians between 6 to 7°C.
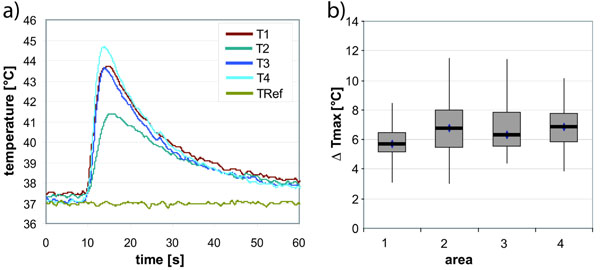
Typical temperature record (a) showing the short temperature peak at four thermocouples around the pin (T1-4) and the reference measurement 2 cm from the insertion point (TRef). Results of the measurements are displayed as box blot diagram of the maximal temperature differences (b) for each area in the femur (area 1) and tibia (area 2-4).
Animal Model
All surgeries went well without serious complications. Implants were correctly placed at the first attempt and were seated firmly within the drill hole. Two Ti-PLA implants slipped into the bone marrow as the screw cap was tightened. They were replaced with new implants in a new drill hole. The ultrasonic equipment was user friendly, although its size was too large compared to the small implants. The time of insertion was kept as short as possible to avoid heat damage to the tissue. In the cortical bone, small portions of the liquefied polymer pushed back to the cortical surface forming a small cap around the implant. Animals recovered quickly and showed normal behavior.
Macroscopic and Radiographic Evaluation
All implants were firmly seated within the bone. Signs of inflammation were never noticed. Postoperative radiographs demonstrated correct placement of the implants. Radiographs after sacrifice showed implants still in place in 2 and 6 months group. At 2 months a radiodense seam with an average of 0.5 mm was clearly visible in the cancellous bone that was even more pronounced at 6 months. In cortical bone a mixture of slightly decreased and increased radiodensity was observed around the implants. Only one PLA implant (cancellous bone site at 6 months) showed a noticeable less radiodense zone.
Histology
The areas of radio dense material corresponded exactly with bone substance stained with toluidine blue, thus confirmed calcification of the newly formed bone. The melted polymer was pressed into the cavities of the cancellous bone, or into the threads of the cortical bone, while implants remained intact. Signs of polymer degradation could rarely be seen at 6 months. Immediate contact between implant and bone matrix was recorded without an interface membrane. New bone formation directly attached to the implant surface and ongoing remodeling was found in all histology sections, which was limited to a distance of 1 mm around the implant. Differences were found in the ratio of bone formation and remodeling between cortical and cancellous bone. In the cancellous bone area new bone formation dominated, which formed a small seam at two months that increased to a uniform bone lamella at 6 months. In cortical areas bone remodeling was more prominent as could be seen in histology sections as small, regularly distributed bone remodeling cavities within the cortex (Figs. 6, 7). The PLA pin, which was noticed in the macroradiographic evaluation, showed bone resorption and infiltration with fibrous tissue at the proximal part of the implant directly underneath the periosteum.
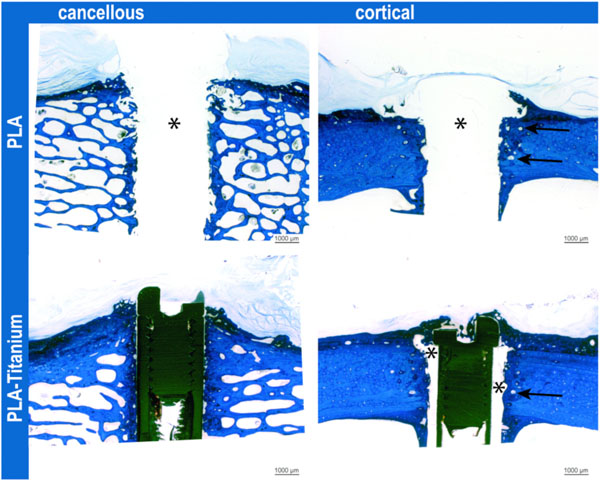
Implants at 6 months in both bone qualities. In cancellous bone, the lamella of new bone at the implant was clearly visible. In cortical bone, widened osteons (arrows) indicated remodeling along the PLA implant (*) or titanium implant with polylactide stripes (*). Toluidine blue stained ground sections.
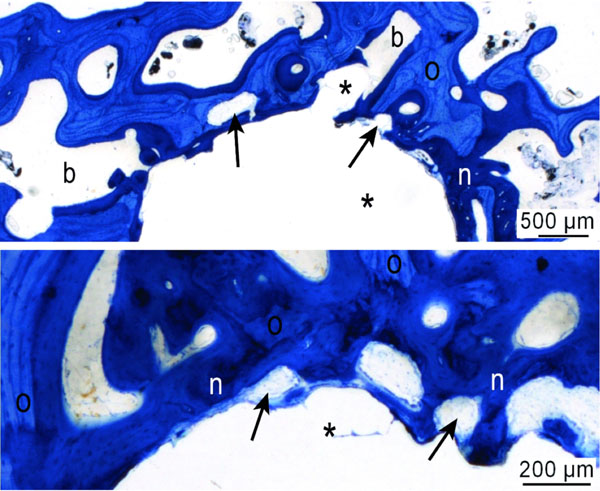
Representative images of new bone formation and remodeling at the PLA implant (*) after 2 months healing period; transverse section. Old bone (O) was partially resorbed and replaced by new bone formation (n, dark stained) by active remodeling units (arrows). Toluidine blue stained ground sections.
Data of histomorphometrical evaluation were supporting the qualitative observation of remodeling in cancellous bone. Values of the sample with proximal bone resorption were in the range of the double standard deviation, and were considered as outliers and excluded from statistical analysis.
For all implant types and observation periods, the bone area near the implant (Sector 1) was higher compared to the more peripheral reference sectors (2 and 3) (Fig. 8). No statistically significant differences of bone formation (measured as area) around the implants were obtained if implant types and observation periods were compared. A tendency was noticed for the inner sector (p = 0.109) and the Ti-PLA implants to show higher values (p = 0.148).
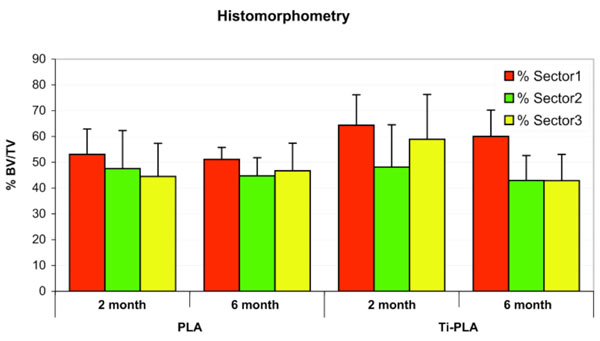
Bone content measurements (total area/bone area) show higher values in the remodeling zone (sector 1) than in the reference zone (sectors 2 & 3). More bone was build up at Titanium-PLA compared to pure PLA pins.
The cellular reaction toward both implant types was identical. At 2 and 6 months, cellular reactions attributed to inflammation were never recorded in both, cortical and cancellous bone. Active osteoblasts lined up as palisades along the newly deposited bone matrix (Fig. 9). Osteoclasts were only found located at the periosteal callus. Their number decreased over time (≤ 8 cells per section at 2 months, ≤ 5 cells per section at 6 months). Few foreign body cells were located directly at the implant (≤ 8 cells per section). Macrophages, lymphoid or plasma cells were rarely seen in the soft tissue adjacent to the bone. Typical signs of active bone remodeling including widening of the Havers’ system were noticed. Bone resorption zone of the single PLA implant sample was filled with loose fibrous tissue, not forming a capsule. Osteoclasts and/or osteoblasts were not present. This indicated that an ongoing process could not be found.
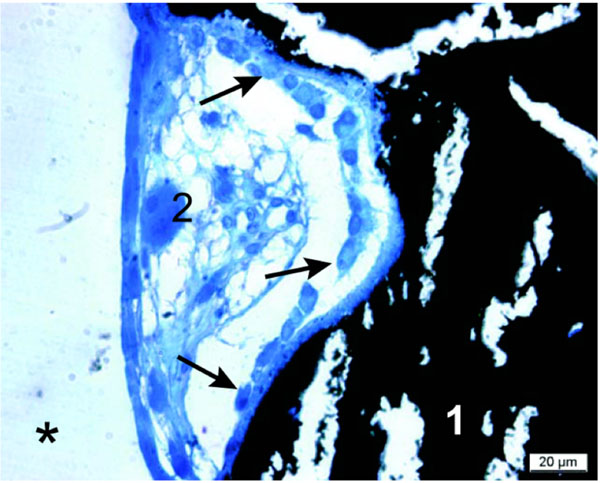
Remodeling unit at the polymer surface (*): Active osteoblasts (indicated by arrows) are lining newly formed calcified bone (1) (crack artifacts by microtome cutting) with a seam of osteoid in between. A single osteoclast was recorded (2). Thin section at 2 months, von Kossa/McNeal staining.
DISCUSSION
In this study it was demonstrated that the BoneWelding® technology was suitable to insert implants consisting of thermoplastic polymer (PLA) into bone. The temperature increase during application of the ultrasound energy and melting of the implant surface did not cause an inflammatory reaction or hamper bone remodeling. Furthermore, this technology could be also successfully used on titanium-PLA implants with excellent bone healing results.
Results of the in vitro temperature measurements demonstrated a transient temperature increase of 6-7°C. This observation indicates a generally lower thermal dose compared to routine drilling procedures in bone [6, 7]. Regarding the good results of histology, the effect of the temperature increase during insertion was below the 45°C threshold for bone tissue injury [16-18] or small enough to be compensated by the repair potential of bone [19]. Therefore, possible damage to the adjacent bone and soft tissue was considered to be negligible for the implantation success.
Overall, the animal model served well for testing of implant biocompatibility, tissue damage due to generation of heat during ultrasound application in cancellous and cortical bone.
The handling of the ultrasonic equipment was easy, even though the ultrasonic device used in this study was relatively large in size in relation to the small implants.
The convincing performance of both implant types could be shown by all results. None of the implants broke, none of the PLA coatings sheared off. PLA melted nicely and penetrated into the bone structures. Absence of signs for polymer degradation were matching with the expected degradation behavior of the PLA, which begins with 6 months [9, 20]. The melting cap at the proximal end of the PLA pin was present throughout all cortical areas. Liquefaction of too much material may have played a role. If less porosity was present as in cortical bone, less material may have intruded into the empty bone spaces and more superfluous material may have accumulated at the cortex. If the implant was not perfectly aligned with the drill hole, more material was “stripped off” and left behind during subsequent insertion. For those areas, exposition of the tissue to the implantation process was comparably high. The melting cap was also effectively shielding the underlying bone from nutrition via the periosteum. But even with this worst case scenario in cortical bone, the results were still very good. In any case, this zone never jeopardized the continuity or quality of bone nor did the cap material negatively influence the insertion quality of the technology. However, an excessive amount of material should be avoided for future application.
Microscopic evaluation of the stained sections revealed excellent biocompatibility of the implants as could be expected considering the type of materials used. New bone formation with direct contact to the implant could always be assessed. Remodeling around the implants was seen in both types of implants. The area close to the implant was filled with more bone compared to non–influenced bone in the periphery, indicating an early adaptation of bone morphology. The bone mass increased visibly in thickness and maturity from 2 to 6 months. Histomorphometry did not confirm these changes. This can be attributed to deviations of the trabecular bone bed or the relatively small sample size in large animal studies. Besides these common constrictions, remodeling and maturation of bone can lead to less bone over time, when initially build up woven bone was replaced by less amount of lamellar bone with higher mechanical strength.
Remodeling activity of cortical bone was not exceeding normal bone healing and was comparable to those observed after normal drilling procedures [21, 22]. Titanium-PLA implants performed better compared to PLA pins. This was not surprising, since osteoblasts are known to attach and proliferate on titanium surfaces [23, 24], which may have resulted in faster deposition of new bone close to the metal part of the implant. Results of the cellular evaluation were in accordance with normal physiologic responses of bone to surgical trauma. Inflammatory cells were not visible and no fibrous interface tissue was grown between implant and bone. The few multinuclear foreign body cells at the polymer surface were not considered as an inflammatory reaction, since no other mononuclear inflammatory cells were present in the environment [25-27]. The single case of bone resorption at the proximal PLA pin was an exception, which cannot be clearly explained or attributed to the insertion method. Since no early stages of soft tissue ingrowth and bone resorption were found in any other sample, further studies need to be carefully evaluated for similar characteristics at early time points.
In conclusion, the BoneWelding® technology proved to be a novel, highly innovative and suitable technology for insertion of implants and repair techniques in orthopedic and trauma surgery. Future studies will have to focus on the biomechanical aspects of implant osseointegration and polymer degradation. Specific implant types and locations in the body system as well as combinations with other types of materials for bone repair will be developed.
ACKNOWLEDGEMENTS
The Study was funded by KTI, the Swiss Commission for Technology and Innovation. Materials were provided by the WW Technology AG, Schlieren, Switzerland. We kindly acknowledge Mr. Urs Mueller, Department of Veterinary Anatomy and Mr. Bruno Gerzner, slaughterhouse of the Vetsuisse Faculty of the University of Zurich, for their help with slaughtering and sample taking.