All published articles of this journal are available on ScienceDirect.
Attachment, Proliferation, and Chondroinduction of Mesenchymal Stem Cells on Porous Chitosan-Calcium Phosphate Scaffolds
Abstract
Symptomatic osteochondral lesions occur frequently, but relatively few treatment options are currently available. The purpose of this study was to conduct a preliminary investigation into a new tissue engineering approach to osteochondral regeneration. The concept is a biphasic construct consisting of a porous, osteoconductive chitosan-calcium phosphate scaffold supporting a layer of neocartilage formed by marrow-derived mesenchymal stem cells. Two experiments were conducted to assess the feasibility of this approach. The first experiment characterized the attachment efficiency and proliferation of primary human marrow-derived mesenchymal stem cells seeded relatively sparely onto the scaffold’s surface. The second experiment compared two different methods of creating a biphasic construct using a much higher density of primary porcine marrow stromal cells. About 40% of the sparsely seeded human cells attached and proliferated rapidly. Constructs formed by one of the two experimental techniques exhibited a layer of cartilaginous tissue which only partially covered the scaffold’s surface due to inadequate adhesion between the cells and the scaffold. This study demonstrates some potential for the approach to yield an implantable biphasic construct, but further development is required to improve cell-scaffold adhesion.
INTRODUCTION
Articular cartilage is the dense connective tissue which covers the ends of bones. Healthy cartilage transmits and dampens joint loads and provides a smooth, almost frictionless bearing surface. Cartilage of the knee is frequently injured, often as a result of sports related trauma, but focal articular cartilage lesions do not heal spontaneously. Articular cartilage is aneural and avascular, and the chondrocytes adjacent to an injury do not proliferate or migrate into the defect [1]. Thus the tissue has very limited capacity for intrinsic repair, and untreated focal cartilage lesions may progress to early osteoarthritis [2]. Furthermore, such defects are associated with pain, swelling, and functional deficit [3, 4].
Focal chondral lesions are a common finding during arthroscopy. A large review of 25,124 knee arthroscopies performed from 1989 to 2004 showed that isolated cartilage lesions occurred in 18% of patients [5]. According to current clinical guidelines, patients with an ICRS grade III or IV chondral lesion who are under 40 years of age may be good candidates for a restorative procedure [6]. Such patients accounted for 7% of all cases in the aforementioned review. Thus grade III and IV focal chondral lesions occur in a sizeable population of relatively young patients who might benefit from hyaline cartilage restoration.
Tissue engineering is an experimental treatment alternative with the potential to regenerate healthy hyaline cartilage tissue, alleviate pain, and restore normal function. The current investigation is related to the biphasic approach to cartilage tissue engineering, in which one layer is designed to support osteogenesis and another to promote chondrogenesis [7-9]. Our goal is to create a bilayered construct which consists of an osteoconductive chitosan-calcium phosphate scaffold underlying a layer of neocartilage formed bymesenchymal stem cells (MSCs) through self-assembly.
Chitosan is a structural analog of glycosaminoglycans (GAGs), and its oligosaccharide degradation products, liberated primarily by enzymatic hydrolysis of the acetylated residues [10], may stimulate the synthesis of GAGs or be directly incorporated into these polysaccharides [11]. Furthermore, chitosan has antimicrobial properties that could reduce the risk of bacterial infection when used as an implant material [12-14]. Combining CaP with chitosan increases its stiffness and osteoconductivity [15]. In addition, the calcium phosphate phase in the composite was found to exist as partially crystalline hydroxyapatite, the mineral phase of bone. Chitosan-CaP scaffolds are approximately 35% porous with pore size ranging from 100-800 μm [15], which is sufficient to support new tissue in growth [16].
Previous investigations of chitosan-CaP scaffolds have focused on their potential application as a bone graft substitute. Human embryonic palatal mesenchymal cells were shown to attach with relatively high efficiency and to proliferate substantially within 7 days [15]. The scaffold was subsequently found to support mineralization by human fetal osteoblasts in vitro and to exhibit biocompatibility and osteoconductivity in vivo in a rat calvarial defect [16]. On the basis of these encouraging results the chitosan-CaP scaffold was selected as the osteogenic layer of our biphasic construct. The purpose of this investigation was to quantify attachment and proliferation of marrow-derived MSCs, as well as to compare two methods of creating a biphasic construct consisting of a chitosan-CaP scaffold supporting an overlying layer of cartilage.
METHODS
Scaffold Fabrication
Cylindrical chitosan-CaP scaffolds (6 mm diameter × 7 mm in height) were prepared as previously described [15], with two important modifications. The first modification was the type of chitosan. In this study the chitosan’s degree of deacetylation (DDA) was 78.7% (Vanson Halosource, Redmond, WA), whereas the DDA was 92.3% in the previous study. Also, scaffolds were subjected to the additional processing steps off reezing at -20°C followed by lyophilization before undergoing ethylene oxide gas sterilization. Freeze-drying has been shown to increase the scaffold’s surface area, porosity, and protein loading capacity [17]. In addition, the scaffolds were not hydrated in culture medium prior to cell seeding in order to take greater advantage of the cationic nature of chitosan for promoting cell adhesion.
Human Mesenchymal Stem Cell Culture
Cell attachment and proliferation were quantified using primary human marrow mesenchymal stem cells. Passage 1 frozen cells from a 24-year old male donor were obtained from the Texas A&M Health Science Center College of Medicine Institute for Regenerative Medicine at Scott & White (Temple, TX). Screening tests verified that these cells met the minimal criteria which define human mesenchymal stem cells as established by the Mesenchymal and Tissue Stem Cell Committee of the International Society for Cellular Therapy [18]. Cells were thawed into StemLife™ MSC Medium (Lifeline Cell Technology, Frederick, MD) and plated at approximately 5×103 cells/cm2. They were subcultured using trypsin before reaching confluence.
Fourth passage cells were suspended at 5×105 cells/ml and 50 µl of this suspension was pipetted onto the top surface of each scaffold. Three additional 50 µl aliquots were frozen and later used to determine the total number of cells seeded onto each scaffold. Cells were allowed 30 min to attach, at which time the scaffolds were flooded with defined chondrogenic medium, or DCM. DCM consisted of high glucose DMEM containing 1% ITS+Premix (BD Biosciences, San Jose, CA), 0.1 mM dexamethasone, 50 µg/mL ascorbate-2 phosphate, 1 mM sodium pyruvate, 40 µg/mL L-proline, 1% antibiotic-antimycotic solution (Sigma-Aldrich, St. Louis, MO), and 10 ng/ml human recombinant transforming growth factor-β3 (PeproTech, Rock Hill, NJ) [19]. Constructs were cultured for 1 or 28 days. Day 1 cultures were used to assess cell attachment quantitatively by measuring DNA (n = 3) and qualitatively using scanning electron microscopy (n = 1). Day 28 cultures were used to evaluate cell proliferation based on content of DNA (n = 6). One Day 28 construct was also examined using SEM.
Quantification of DNA
Day 1 constructs were rinsed with phosphate buffered saline (PBS) and incubated with 0.25% trypsin. Cells released using trypsin and those which had been frozen were lysed in 0.1% Triton X-100, 5 mM Tris-HCl, 20 mM EDTA. Cell lysate was added to TNE buffer containing 0.2 µg/ml Hoechst 33258 and fluorescence intensity measured in a GloMax®-Multi Jr (Promega Corporation, Madison, WI). DNA content was determined from a standard curve created using calf thymus DNA. Cell seeding efficiency was calculated as the ratio of cells recovered from the scaffold to the total number which had been seeded. Day 28 constructs were digested in 1% papain for 24 h at 60°C according to Hoemann [20]. DNA content of each papain digest was measured by the Hoechst assay as described above. Population doubling time Td was calculated from Equation 1, where ti and tf were the initial and final time points, respectively, at which DNA measurements qf and qi were made.
Scanning Electron Microscopy
Constructs for SEM were fixed in 2.5% glutaraldehyde in PBS, dehydrated in graded ethanol and hexamethyldisilazane, and air dried. They were sputter coated with platinum and imaged using a JEOL JSM-6500F Field Emission Scanning Electron Microscope.
Creation of Biphasic Constructs
Long term studies to evaluate different techniques of creating biphasic constructs were performed using primary porcine marrow mesenchymal stem cells. Four femurs from two pigs were obtained from a local meat processor. Marrow and fat from the diaphyseal region were collected into 50 ml centrifuge tubes and the marrow isolated by spinning at 1000g for 10 min. Pooled marrow was disaggregated by repeated pipetting and plated into a standard T-175 flask in Dulbecco’s Modified Eagle Medium (DMEM) containing 10% fetal bovine serum. Medium was changed after 24 h and the flask rinsed twice with PBS to remove non-adherent cells and debris. The cells were subcultured using trypsin upon reaching 90% confluence.
Biphasic construct technique #1 (Fig. 1): Molten 2% low gelling temperature agarose was poured over the scaffolds until they were buried to depth of 5 mm. Once the agarose had solidified a biopsy punch was used to cut a cylinder above the scaffold. The overlying agarose was removed by Pasteur pipette under vacuum. Second passage cells were suspended in expansion medium at 1.5×108cells/ml, and a 50 µl aliquot of the suspension pipetted onto each scaffold (7.5x106 cells/scaffold). The cells were allowed to settle for 60 min before the wells were flooded with DCM. Two days after cell seeding the agarose was removed to facilitate transport of nutrients and waste products.
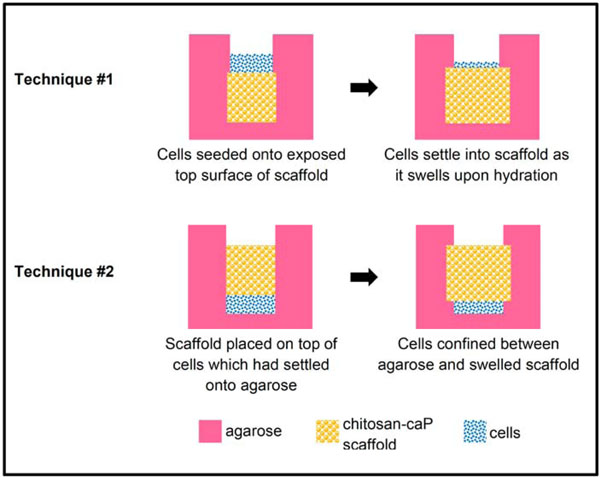
Schematic diagram of techniques for high density seeding of MSCs onto porous chitsosan-CaP scaffolds.
Biphasic construct technique #2 (Fig. 1): Molten 2% agarose was poured around stainless steel rods of the same diameter as the scaffolds. The rods were removed after the agarose had gelled and the bottom of the holes also covered with a thin layer of agarose. The same number of cells as in Technique #1 were pipetted into each hole and allowed to settle for 30 min. Scaffolds were inserted into the holes and pressed gently against the cells. The wells were then flooded with DCM. Ten days after cell seeding three of the constructs were pulled out of their agarose holes. However, it was apparent that most of the cap of cells protruding above the scaffold’s surface were lost in the process. Therefore the other three scaffolds were left in their inverted configuration for the remainder of the experiment. In both techniques, medium was replaced every 4-5 days.
Assessment
Gross inspection revealed that the only constructs which displayed visible cartilaginous tissue were the three Technique #2 scaffold which had been allowed to remain in their original configuration for the entire culture duration. Therefore these were the only constructs which were subjected to histological and biochemical characterization. A piece of tissue approximately 1 cubic millimeter in size was removed from each construct and digested in papain. Glycosaminoglycan (GAG) content was determined using the dimethyl methylene blue (DMB) assay. Digest was added directly to a DMB assay buffer prepared according to Hoemann [20] and the optical density at 530 nm (OD530) was read using a μQuant spectrophotometer (Bio-Tek Instruments, Winooski, VT). The OD590 was also read and subtracted from the OD530 reading to increase sensitivity. GAG content was calculated from a standard curve created using chondroitin sulphate. DNA contentof the same digest was determined by the Hoechst assay as described above.
The three Technique #2 scaffolds from which tissue had been sampled for biochemistry were fixed in 10% neutral buffered formalin, embedded in paraffin, and sectioned for histology and type II collagen immunohistochemistry. Sections for histology were stained with 2% toluidine blue to detect proteoglycan. Sections designated for immunohistochemistry were incubated in hyaluronidase (2 mg/ml in tris-buffered saline) for 30 min at 37°C followed by pronase (0.5 mg/ml in PBS) for 10 min at 37°C for antigen retrieval. The primary antibody was II-II6B3 (undiluted supernatant) from the Development Studies Hybridoma Bank (University of Iowa, Iowa City, IA). Sections were incubated in primary antibody for 2 h at room temperature. The rest of the staining was performed using the SuperPicture™ 3rd Gen IHC Detection Kit (Life Technologies, Grand Island, NY) according the manufacturer’s instructions. Stained sections were imaged on a Leica DM2500 microscope equipped with Leica DFC420 C digital camera.
One Technique #1 construct was fixed in glutaraldehyde and examined using SEM in the same manner as described for the proliferation experiment.
RESULTS
The mean 30 min seeding efficiency of human bone marrow MSCs on chitosan-CaP scaffolds was 37.5%, 95% confidence interval (32.47 - 47.70). At 24 h SEM showed that the cells had a flattened and elongated or stellate morphology, and some cells spanned the gap between neighboring chitosan-CaP beads (Fig. 2). On average the DNA content increased almost 50-fold over the 28 d culture period, which translates to a mean population doubling time of 4.84 d, 95% confidence interval (4.71 - 4.96).
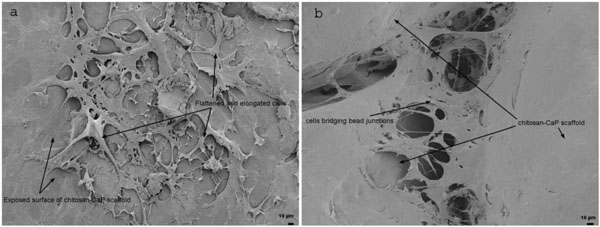
Scanning electron micrographs of human MSCs on CHI-CaP at 24 h. a - face of bead (200X); b - junction between beads (250X).
Technique #1 failed to form a macroscopically evident layer of tissue atop the porcine bone marrow MSC/chitosan-CaP constructs at 28 d. However, SEM revealed that the scaffold’s surface was largely covered with a thin sheet of connective tissue (Fig. 3). Only in the crevasses formed at the junctions between chitosan-CaP beads were there some isolated cells with the more rounded shape characteristic of chondrocytes. Examination of the tissue-scaffold interface demonstrated a tight junction with the tissue in direct contact with scaffold’s surface (Fig. 4). The constructs formed by Technique #2 which were pulled out of the agarose at 10 d also did not contain any macroscopic neotissue. Those which were left in their original configuration retained some neotissue on the surface which was cartilage-like in appearance, but it did not cover the majority of the surface (Fig. 5a). The GAG content of this tissue was 247.2 ng GAG per ng of DNA, 95% confidence interval (241.2 - 253.01). Assuming 7.7 pg DNA/cell [21], the tissue contained approximately 2 ng GAG/cell. Cells were round, located within lacunae, and surrounded by extracellular matrix which exhibited metachromatic toluidine blue staining (Fig. 5b). The extracellular matrix stained intensely for collagen type II (Fig. 5c). Some separation was noticed between the tissue and the scaffold, and this is likely attributable to shrinkage of the scaffold during processing.
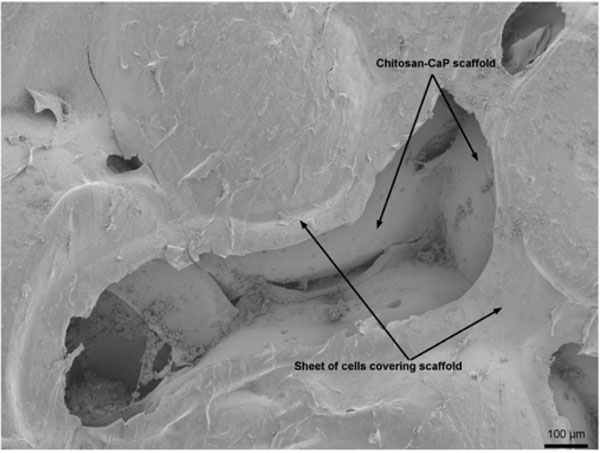
Scanning electron micrograph of human MSCs seeded at low density on CHI-CaP and cultured for 28 d in chondrogenic medium (85X).
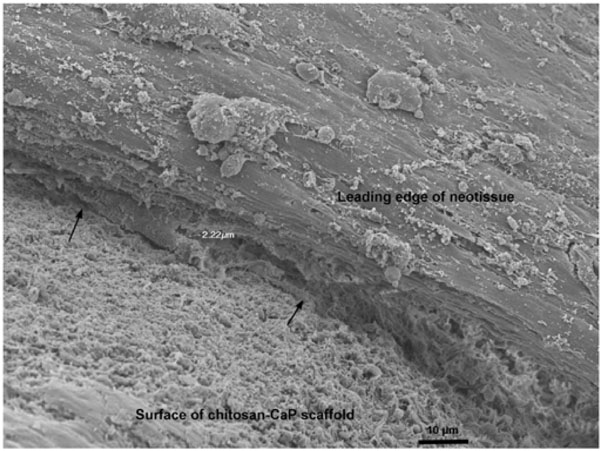
Scanning electron micrograph of MSC/chitosan-CaP scaffold interface after 28 d culture in chondrogenic medium (1000X). Arrows point to MSC-scaffold interface.
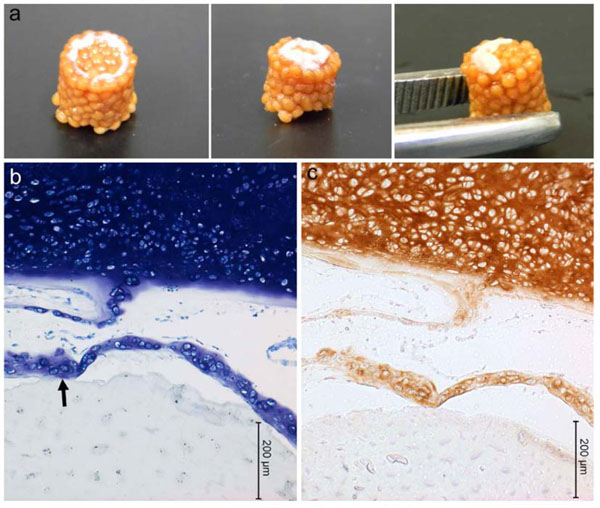
Porcine mesenchymal stem cells cultured on chitosan-CaP scaffolds for 28 d in chondrogenic medium. a – gross appearance of constructs formed by Technique #2 (refer to text); b – toluidine blue staining of histological sections (arrow points to scaffold-tissue interface); c – positive immunostaining for type II collagen as indicated by brown DAB chromagen.
DISCUSSION
Articular cartilage provides smooth, lubricated joint surfaces for continuous gliding motion, absorbs mechanical shock, and distributes load to bone. However, it is susceptible to injury through a number of mechanisms, including trauma (e.g., patellar dislocation or hyperflexion), osteochondritis dissecans, osteonecrosis, and congential anatomical abnormality. When an injury is sustained, articular cartilage has a very limited capacity for intrinsic healing. Because the tissue is avascular, the injury does not provoke the typical wound healing response characterized by inflammation and invasion of undifferentiated mesenchymal cells. Therefore injuries to articular cartilage often progress to severe degenerative osteoarthritis, treatment for which is frequently total joint replacement.
The current study is a preliminary proof-of-concept investigation of a new tissue cartilage tissue engineering approach involving high-density seeding of mesenchymal stem cells onto a porous chitosan-CaP osteoconductive scaffold. In clinical practice autologous mesenchymal stem cells isolated from a bone marrow or adipose tissue biopsy would be expanded in monolayer and combined with porous chitosan-CaP so as to form a scaffold-free layer of cartilage interdigitating with the pores of the scaffold. This construct would be implanted in a manner similar to an osteochondral allograft. Based on the scaffold’s previously measured compressive stiffness of approximately 10 MPa [15], it would facilitate transmission of joint forces to underlying bone. Previous research involved implantation of non-freeze dried scaffolds made from 92.3% DDA chitosan into rat calvarial defects [16]. Although the scaffolds did not substantially degrade within 12 weeks, they became enclosed by a thin layer of new bone which was continuous with native bone in some areas. Histology revealed new bone formation in direct contact with the scaffold. The slow rate of degradation was attributed mainly to the chitosan’s high DDA, and the lower DDA chitosan used in the current formulation could be expected to degrade at a faster rate [22], allowing infiltration by new bone. Higher porosity created by freeze drying the scaffolds can also be expected to accelerate degradation. An in vivo investigation is underway to determine whether the modified scaffold will be resorbed and replaced by host bone after implantation in a joint.
The bilayered approach to cartilage tissue engineering, with one layer designed to support osteogenesis and another to promote chondrogenesis, has previously been presented [8,9]. However, the bilayered construct typically contains two different types of scaffold material fused together or different types of cells seeded into the top and bottom halves of the scaffold. The bilayered construct presented herein consists of an osteoconductive scaffold and a layer of neocartilage formed by mesenchymal stem cells through self-assembly. Waldman et al. devised a similar strategy using articular chondrocytes layered on top ofa porous calcium polyphosphate substrate [23].
The osteoconductive scaffold is fabricated from a mixture of chitosan and calcium phosphate precipitated together into small beads. Porosity is created when the beads are fused together by briefly immersing inacetic acid, a process analogous to sintering of metal balls. It is therefore a simple matter to fabricate custom scaffolds by fusing the beads together when they are packed into a negative mold of the desiredshape. This study demonstrates that freeze-dried chitosan-CaP scaffolds support cell attachment and proliferation of primary human bone marrow MSCs. Within 24 h the cells had flattened against the scaffold’s surface and acquired a spindle-like morphology, which is characteristic of attached mesenchymal stem cells with osteogenic and chondrogenic potential [24]. The seeding efficiency of about 38% is consistent with that previously reported for human embryonic palatal mesenchymal cells on scaffolds with the same composition [15]. Cell proliferation was also similar between the two studies. The DNA of human embryonic palatal mesenchymal cells increased approximately 3-fold from Day 3 to Day 7, and our study shows that proliferation is sustained up to an increase of approximately 50-fold (mean population doubling time under 5 days). Furthermore, this proliferation occurred in serum-free defined chondrogenic medium.
This study also demonstrates that a partial layer of scaffold-free cartilage can be formed atop a porous chitosan-CaP scaffold by seeding at very high cell density. For all three of the Technique #2 scaffolds allowed to remain in their original configuration, cells which amassed near the surface and were able to undergo chondrogenic differentiation and form a cartilage-like tissue. Unfortunately attachment between the scaffold and the neotissue was poor, and the technique yielded incomplete tissue coverage of the scaffold’s flat surface. Nonetheless, Technique #2 was superior to Technique #1 for which 6 out of 6 scaffolds yielded no macroscopically evident tissue formation. We attribute this phenomenon to the migration of the freshly seeded cell aggregate into the bulk of the scaffold as it expanded laterally during rehydration. Whereas a layer of cells underneath the Technique #2 scaffolds was clearly visible 24 h after cell seeding and thereafter until 28 d, the layer of cells resting on the Technique #1 scaffolds’ surfaces immediately after seeding was no longer visible after 24 h.
The potential advantage to Technique #1 is direct contact between the cells and the bulk of the culture medium, which facilitates nutrient and waste transport. The disadvantage is that cells seeded onto a dry scaffold do not remain in their original position as the scaffold expands during rehydration. The advantage to Technique #2 is that the cells remain at the surface as the scaffold expands laterally during rehydration. Confinement of the cells between the scaffold and the agarosemay also serve a stimulus for cartilaginous extracellular matrix organization [25]. The potential disadvantage is that nutrients in the culture medium must travel through the scaffold or agarose to reach the cells.
Although extracellular matrix production may have been hindered by insufficient supply of nutrients, we believe that the main hindrance to formation of a continuous cartilage layer by Technique #2 was inadequate cell-scaffold adhesion. In a previous study, the attachment efficiency of human embryonic palatal mesenchymal stem cells on similar chitosan-CaP scaffolds achieved a maximum of approximately 60% after 2 h [15]. Cell attachment which promotes growth of the tissue into the pores of the scaffold is essential for creating a mechanical interlock between the tissue and the scaffold which will prevent the tissue being dislodged under joint loading. One strategy to improve tissue-scaffold integration involves precoating the scaffold with an extracellular matrix protein to which cell binding is mediated by various integrin receptors. In particular, a collagen type I substrate has been shown to enhance MSC adhesion and proliferation [26]. Preliminary investigationsin our laboratory have shown that coating the scaffold with collagen greatly enhances cell adhesion and promotes formation of a uniform layer of cartilage in the area of cell seeding. Our results demonstrate the feasibility of a biphasic MSC/chitosan-CaP construct which might be further developed into a tissue-engineered alternative for chondral lesion repair.
CONFLICT OF INTEREST
The authors confirm that this article content has no conflict of interest.
ACKNOWLEDGEMENTS
Research reported in this publication was supported by the National Institute of Arthritis and Musculoskeletal and Skin Diseases of the National Institutes of Health under Award Number 1R15AR057934-01A1. The content is solely the responsibility of the authors and does not necessarily represent the official views of the National Institutes of Health. Some of the materials employed in this work were provided by the Texas A&M Health Science Center College of Medicine Institute for Regenerative Medicine at Scott & White through a grant from NCRR of the NIH, Grant # P40RR017447. The II-II6B3 monoclonal antibody developed by T. F. Linsenmayer was obtained from the Developmental Studies Hybridoma Bank developed under the auspices of the NICHD and maintained by The University of Iowa, Department of Biology, Iowa City, IA 52242.