All published articles of this journal are available on ScienceDirect.
Osteogenic Predifferentiation of Human Bone Marrow-Derived Stem Cells by Short-Term Mechanical Stimulation
Abstract
It is commonly accepted that bone marrow-derived stem cells (BMSCs) have to be expanded in vitro, but a prolonged time in culture decreases their multilineage potential. Mechanical and biological stimuli have been used to improve their osteogenic potential. While long-term stimulation has been shown to improve osteogenic differentiation, it remains to be seen whether short-term stimulation is also sufficient.
We investigated the influence of 24 hours' cyclic loading (0.05Hz, 4kPa) on gene expression of human BMSCs in three-dimensional fibrin-DMEM constructs (n=7) in a compression bioreactor using DNA-array technology. Expression of the following genes showed a significant increase after mechanical stimulation: 2.6-fold osteopontin (OPN) and integrin-β1 (ITGB1), 2.2-fold transforming growth factor-β-receptor 1 (TGF-β-R1) and 2.4-fold SMAD5 expression, compared to controls without mechanical stimulation (p<0.05 each). Platelet-derived growth factor-α (PDGF-α ) and annexin-V were also significantly overexpressed, the mechanical stimulation resulting in a 1.8-fold and 1.6-fold expression (p<0.05).
Cells were identified as osteoblast precursors with a high proliferative capacity. Given the identical in-vitro environment for both groups, the increase in gene expression has been interpreted as a direct influence of cyclic mechanical stimulation on osteogenic differentiation. It may be postulated that short-term mechanical stimulation results in an improved osseous integration of tissue engineered grafts in bone defect healing.
INTRODUCTION
Major bone defects remain an unsolved therapeutic problem in orthopaedic surgery, especially in revision arthroplasty, treatment of pseudarthrosis or after tumour resections [1]. The use of autologous and allogenic bone grafts is the most widely used regenerative approach [2]. Fundamental differences in biology have been noted in these two approaches: Allografts demonstrate only osteoconductive capacity with low overall integration and an unsatisfactory long-term clinical outcome [3-5]. Autografts show osteoinductive and osteogenic characteristics, leading to significantly better clinical results [6]. However, their use is limited by restricted availability and significant donor-site morbidity [7].
A therapeutic approach that harnesses the osteogenic potential of autografts, without the limitations of low availability and high donor-site morbidity is still missing in the treatment of bone defects.
Therefore, autologous osteogenic cells like periosteum-derived cells in different matrices have been investigated in regenerative bone defect therapy and promising results have been published [8, 9]. Bone marrow-derived stromal cells have also been investigated in animal and clinical trials, an accelerated healing of bone defects being reported [10-13].
BMSCs have little donor-site morbidity and are easily expanded in vitro [14], but their multilineage potential and proliferative capacity decreases with time in vitro [15]. It has therefore been recommended to keep the in-vitro phase as short as possible in clinical situations [16, 17]. A predifferentiation of cells during the in-vitro period has been reported to be beneficial, as the osteogenic potential of BMSCs can be augmented through biological or mechanical stimulation lasting for several weeks [18, 19].
In the present study we wanted to investigate whether a short-term mechanical stimulation of just 24 hours is sufficient to achieve an osteogenic predifferentiation in vitro. A short period of stimulation and thereby short in-vitro time might be advantageous for subsequent in-vivo use. Maintaining the high proliferative capacity and directing the cellular differentiation into an osteogenic lineage may improve results of cell-based approaches in the treatment of bone defects.
MATERIALS AND METHODOLOGY
Study Design
BMSCs were harvested from seven different donors, all cells then being expanded separately in cell-culture flasks. After a sufficient amount of cells had been achieved, they were transferred into three-dimensional constructs consisting of an elastic fibrin-DMEM matrix and then put between two slices of freeze-dried cancellous bone. All constructs were placed in bioreactors, as described previously [20]. Half of the constructs underwent cyclic mechanical compression; the other half remained without mechanical stimulation as a control. The gene expression in mechanically stimulated cells was normalized versus unstimulated controls.
Cells and Cell Culture
The BMSCs were gained from aspirates of the proximal femur during total hip arthroplasty operations. All 7 donors (4 female, 3 male, average age 61 years) gave informed consent. A pre-existing bone disease was excluded anamnestically and by bone densitometry investigation (Lunar-DPX, USA). Approximately 5ml bone-marrow aspirate were harvested from the intertrochanteric region of the proximal femur, and kept at 8°C until further processing within 4 hours.
Density centrifugation was performed (Histopaque 1.077g - Sigma-Aldrich, Germany) for 15 minutes at 1500rpm. The interphase containing the BMSCs was transferred into culture medium (DMEM + 10% FCS [Biochrome, Germany] + 100U/ml penicillin + 100µg/ml streptomycin [Sigma-Aldrich, Germany]). The cells were dispersed in culture flasks (Falcon, Greiner, Germany) and then incubated at 37°C and 5% CO2. Passage into a new flask was performed at approximately 70% confluence.
3D Matrix/Bone-Fibrin-DMEM Constructs
After having reached passage 3 in the above given cell culture medium, cells were transferred into 3D constructs, consisting of a fibrin-DMEM-mix matrix and placed between two slices of freeze-dried human cancellous bone. Every construct contained 1x106 cells in 600µl DMEM + 10%FCS (Biochrome, Germany) + 100U/ml penicillin + 100µg/ml streptomycin (Sigma-Aldrich, Germany) + 2.4% aprotinin (Bayer, Germany). For polymerization 30µl fibrinogen and 1IU/ml thrombin (Aventis, Germany) were added. The matrices hardened for one hour at 37°C at 5% CO2, before being transferred into the bioreactors.
The freeze-dried cancellous bone slices measured 15mm in diameter and 4mm in depth. Prior to construct mounting, they were rehydrated for two hours in culture medium.
Mechanical Stimulation in the Compression Bioreactor
The principle of the compression bioreactor (Fig. 1) has been described previously [20]. A closed cylindrical polyethylene chamber communicates with the incubator via four sterile filters (0.2µm pore diameter). The cell-matrix constructs were mechanically stimulated between two flexible silicon membranes. While the upper membrane loads the construct (Fig. 2) perpendicularly to its surface, the lower membrane transfers the pressure via a standard infusion tube to a pressure transducer.
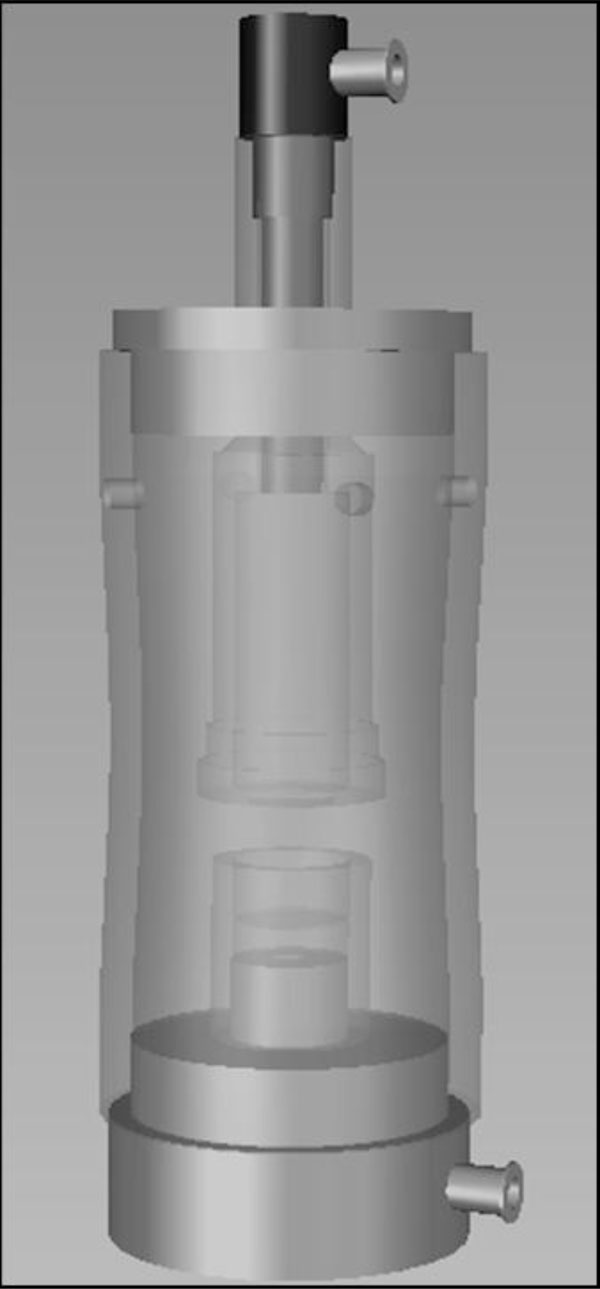
Compression bioreactor for cyclic loading of the constructs under sterile culture conditions.
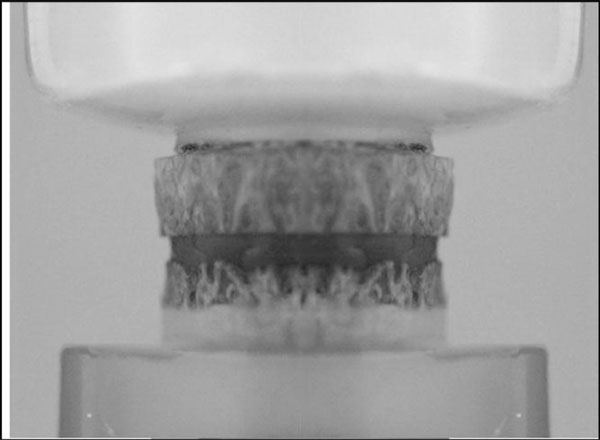
Construct with BMDSCs in a fibrin matrix.
In the present experiment, we applied a cyclical pressure of 4kPa, resulting in a deformation of approximately 1mm (Δh/h=25%), at a frequency of 0.05Hz. The loading environment of the lower extremities during physiological activities such as walking and running is cyclic [21], and the frequency used in our experiment has been described to be within physiological conditions [22]. Control constructs (n=7) were cultivated in bioreactors without mechanical stimulation. After 24 hours, the cyclic compression was terminated and the constructs were harvested.
RNA Extraction and Gene Expression
RNA isolation was started within two minutes. Under sterile conditions the fibrin-DMEM matrices were harvested from the compression bioreactors: the matrix was dissected sharply from the cancellous bone and transferred immediately into 2ml RNA-extraction solution (Trifast, Peqlab, Germany). Matrices were homogenized mechanically (Ultra-Turrax-T8, IKA, Germany) directly afterwards. The RNA extraction was performed according to the protocol of the supplier. RNA integrity was controlled in a Bio Sizing Assay (Eukaryote Total RNA Nano, Agilent Technologies 2100 Bioanalyzer, Germany). After measurement of total RNA using a photometer, the RNA was stored at -140°C until further use.
The expression of osteogenesis-specific genes was investigated using the GEArray-Q-Series Human Osteogenesis Gene Array (Biomol, Germany). 3-3.5µg total RNA was used for each array. cDNA synthesis was accomplished at 37°C for 25 minutes. The subsequent labelling was carried out with Biotin-16-dUTP (Roche, Germany) and an amplification labelling kit (Biomol, Germany) according to the protocol of the supplier. 30 cycles of PCR amplification were implemented, with 85°C, 50°C and 72°C for 1 minute each (MJ Research Inc, USA). Hybridisation of the PCR product on the arrays was carried out at 56°C for 12 hours. Unspecific binding had been blocked by prehybridisation with salmon-sperm DNA (Roche Diagnostics, Germany) for 2 hours. After labelling with streptavidin-biotin antibodies and addition of CDP-Star solution (Biomol, Germany), the array signal was transmitted onto x-ray films (Kodak, Germany). Films were developed and scanned. The gene expression was analyzed using the programs ScanAlyze Version 2.5 (Eisen Software, USA) and GEArray Analyzer 1.3 (Superarray, USA). Following the recommendation of the array supplier, genes with unreliably low signals were excluded from further analysis.
STATISTICS
Statistical analysis was performed using SPSS 12.0. After background subtraction, the signals were normalized to the GAPDH as house keeping gene. The evaluation was carried out comparing directly the expression of mechanical stimulated cells versus controls of one donor. The expressions of the controls were defined as 1. All experiments were done in triplicate for all the 7 patients, all samples were subjected to DNA array analysis. A Wilcoxon signed rank test was carried out for statistical testing, a level of significance of p=0.05 was chosen.
RESULTS
The RNA isolation was successful in all cases; all specimens could be included in the data analysis (Table 1). We noted a strong expression of core-binding-factor-1 (Cbfa1) in all BMSCs, showing no significant difference between the two treatment groups (p=0,463). We found significant differen-ces in the expression of mechanically stimulated BMSCs compared to controls, as shown in Fig. (3).
Mean Changes in mRNA Expression of BMDSCs Through 24h of Mechanical Stimulation Normalized Versus Control Group w/o Mechanical Stimulation Measured with a Human Osteogenesis Gene Array (Biomol, Germany)
Gene | Xfold Increase | Gene | Xfold Increase | Gene | Xfold Increase |
---|---|---|---|---|---|
alk. phosphatase | 0 | COL7A1 | 0 | SMAD9 | 1.5 |
annexin v | 1.6 | COL9A2 | 1.3 | MMP10 | 1.3 |
ARSE | 0 | GM-CSF | 0 | collagenase-3 | 0.9 |
Osteocalcin | 0 | G-CSF | 0.3 | gelatinase A | 0.9 |
Biglycan | 1.6 | CTSK | 1.1 | MMP8 | 0.1 |
BMP1 | 0 | Decorin | 1 | gelatinase B | 1 |
BMP2 | 0 | EGF | 0 | Hox7 | 0.6 |
BMP3 | 0.1 | EGFR | 1.1 | MSX2 | 0 |
BMP 4 | 0 | FGF1 | 0.1 | NFkB | 0.4 |
BMP5 | 0 | FGF2 | 0 | PDGFa | 1.8 |
BMP 6 | 0 | FGF3(int-2) | 0 | CBFA1 | 1 |
BMP7 | 0 | FGFR1 (FLG) | 0.5 | CBP1 | 0.9 |
BMP 8 | 0.6 | FGFR2 | 0 | CBP2 | 0.1 |
ALK-3 | 0.6 | FGFR3 | 0.2 | SOX9 | 0 |
CASR | 0.1 | FLT1 | 0 | SPARC cleav. prod. | 1.1 |
CD36 | 0.5 | fibronectin-1 | 0.8 | OPN | 2.6 |
CD36L1 | 1.3 | BMP3B | 0 | TGFb1 | 0 |
CD36L2 | -0.1 | ICAM-1 | 0 | TGF b2 | 0 |
COL10A1 | 0.3 | IGF-1 | 0.9 | TGF b3 | 1.4 |
COL11A1 | 1.7 | IGF-1R | 0 | TGFBR1 | 2.2 |
COL12A1 | 1.1 | IGF-II | 0 | TGFbR2 | 0.3 |
COL14A1 | 0.8 | Integrin a1 | 1.5 | TNFa | 1.5 |
COL15A1 | 0 | Integrin a2 | 0.7 | TWIST | 0.3 |
COL16A1 | 1.3 | Integrin a3 | 1.3 | VCAM-1 | 0.1 |
COL17A1 | 0.3 | Integrin aM | 0 | VDR | 0 |
Endostatin | 0.6 | Integrin aV | 0.3 | VEGF | 0 |
COL19A1 | 0 | Integrin b1 | 2.6 | VEGF-B | 1.3 |
COL1A1 | 0 | SMAD1 | 0.9 | VEGF-C | 1.3 |
COL2A1 | 1.1 | SMAD2 | 1.1 | pUC18 | 0.1 |
COL3A1 | 1.4 | SMAD3 | 1.3 | 0 | 0 |
COL4A3 | 0 | DPC4/SMAD4 | 0.4 | GAPDH | 1 |
COL4A4 | 0 | SMAD5 | 2.4 | cyclophilin A | 2.3 |
COL4A5 | 0 | SMAD6 | 0 | RPL13A | 0.9 |
COL5A1 | 1.1 | SMAD7 | 0.8 | b-actin | 0.6 |
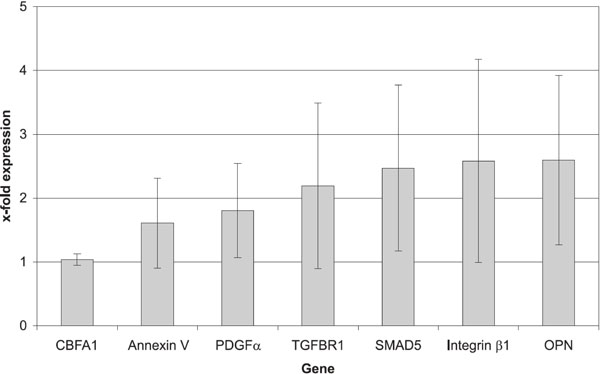
Gene expression of mechanically loaded constructs normalized vs control constructs without mechanical stimulation.
Matrix Proteins
A significant difference was noted for the expression of the osteopontin (OPN) gene, which showed a 2.6-fold expression in mechanically stimulated cells, compared to controls (p=0.043).
Cell-Surface Receptors
Furthermore, we found a 2.6-fold increase in the expression of the integrin-β-1 gene and a 2.2-fold increase in the expression of the transforming-growth-factor-beta-receptor 1 (TGF-β-R1), both differences being significant (p=0.018).
Intracellular Signalling Molecule
The mechanical stimulation also had a direct effect on the expression of an intracellular signal molecule, the SMAD-5 gene, which showed a 2.4-fold increase (p=0.028).
Moreover, we found significant increases of two other genes: 1.8-fold for platelet-derived growth factor-alpha (PDGF-(α ) (p=0.027) and 1.6-fold for annexin-V (p= 0.034).
DISCUSSION
BMSCs show high proliferative capacity and do not undergo terminal osteoblast differentiation in vitro without external stimulation. In the present study, we could show for the first time that 24 hours of cyclic compression is able to increase the expression of several osteogenesis-specific genes and thereby lead to an osteogenic predifferentiation of BMSCs.
The gene expression analysis showed a marked expression of Cbfa1, the master control gene of osteoblastic differentiation [23]. The BMSCs shifted to the level of immature osteoprogenitors [24].
By definition, these cells do not yet show expression of the typical markers for osteogenesis, like osteocalcin, alkaline phosphatase or type-I collagen, which were not detectable in our trial. However, they do express the non-collagenous matrix protein osteopontin [25], which was significantly increased by mechanical stimulation in our setting. It might be hypothesized that more BMSCs were directed towards immature osteoprogenitors.
As a non-collagenous protein of the bone matrix, OPN has further influences on bone metabolism: amongst others, it plays an important role during bone remodelling, inflammation and under mechanical stress [25, 26]. An elevated OPN expression has also been noted during the early phase of fracture healing, prior to callus formation. As Kawahata and co-worker hypothesized that it might be a trigger for osteogenesis [27], the increased expression of OPN after 24 hours of cyclic compression may be an indicator of initial osteogenesis.
Another important effect of OPN has been identified in mice: ectopically implanted autologous bone showed lower transplant vascularization and integration, due to reduced bone remodelling [28]. A 2.6-fold increase of OPN expression by mechanical stimulation of constructs with BMSCs might thus augment bone remodelling and improve transplant vascularization and integration.
The mechanically stimulated BMSCs also showed an increased expression of integrin-β 1, a part of transmembrane glycoprotein that forms heterodimers with integrin-α subunits and links the cytoskeleton to the extracellular matrix. The importance of integrin-β1 in osteogenesis and osteoblast function has been shown in mice [29]. Together with the α1-3 subunits, it binds to collagen, and ligand-binding leads to increased expression of osteoblast markers [30, 31]. The β1-subunit has been identified as the most important integrin in the development of BMSCs to osetoblasts [32]. Its increased expression might signalize the promotion of osteogenic differentiation in BMSCs following 24 hours of mechanical stimulation.
In addition, the mechanically stimulated BMSCs showed a significant increase in the expression of the TGF-ß-receptor1 [33]. This receptor has been identified on osteoprogenitor cells and also on terminally differentiated osteoblasts [34]. Its ligand TGF-ß has been shown to be involved in the regulation of proliferation and migration of progenitor cells and leads to an increase of osteoblastic differentiation, independently of their differentiation state [35]. It promotes osteogenic differentiation in BMSCs, with enhanced matrix synthesis and calcification [36, 37]. The mechanically stimulated BMSCs with an increased expression of the TGF-β-receptor1 might therefore be more sensitive to TGF-ß stimuli and show augmented osteogenic differentiation when implanted into bone defects.
The increase of the intracellular signal molecule Smad-5 may be another indicator of osteogenic predifferentiation. Smad-5 interacts with Smad-1 and mediates signals from cytokines of the TGF-ß superfamily, and among them the osteoblastic differentiation of progenitor cells [38, 39]. This may result in a facilitation of osteogenic differentiation of mechanically stimulated cells in bone defects.
The expression of the growth factor PDGF-α also increased following mechanical stimulation. PDGF-α is known to induce osteogenic differentiation, has additional chemotactic effects on osteoprogenitor cells and enhances the proliferation of osteoblasts [40]. These are all effects that may contribute to improved results following mechanical stimulation of BMSCs before using them in cell-based therapies.
Annexin-V gene expression was significantly increased after mechanical stimulation. Annexin-V protein builds Ca2+ channels, leading to mineralization of bone matrix in the growth plates during osteogenesis [41]. An increased expression of annexin-V enhances these processes of mineralization [42]. Such mineralization processes were observed in a prior study, whereas periosteal cells underwent long-term mechanical stimulation in bioreactors [20].
CONCLUSION
In recent years, different methods have been developed to stimulate osteogenic differentiation of BMSCs in vitro an in vivo, like medium supplements, growth factors or viral transfection [1, 43]. The approach of mechanical stimulation presented here is advantageous, because it can be directly transferred to clinical application. The selected fibrin matrix allows temporary fixation of BMSCs in a bone defect and has shown excellent biocompatibility [8, 44].
Our results show a concomitant increase of genes coding for matrix molecules, receptors and growth factors by mechanical stimulation. The presented data indicate that 24 hours are sufficient to obtain changes in gene expression, resulting in an osteogenic predifferentiation of BMSCs. The shift towards immature osteoprogenitor cells combines the desired high proliferative capacity with an osteogenic potential that makes them a promising tool in cell-based treatment of bone defects. It may be postulated that short-term mechanical stimulation will result in an improved osseous integration of tissue engineered grafts in bone defect healing.