All published articles of this journal are available on ScienceDirect.
Carbon Nanostructures in Bone Tissue Engineering
Abstract
Background:
Recent advances in developing biocompatible materials for treating bone loss or defects have dramatically changed clinicians’ reconstructive armory. Current clinically available reconstructive options have certain advantages, but also several drawbacks that prevent them from gaining universal acceptance. A wide range of synthetic and natural biomaterials is being used to develop tissue-engineered bone. Many of these materials are currently in the clinical trial stage.
Methods:
A selective literature review was performed for carbon nanostructure composites in bone tissue engineering.
Results:
Incorporation of carbon nanostructures significantly improves the mechanical properties of various biomaterials to mimic that of natural bone. Recently, carbon-modified biomaterials for bone tissue engineering have been extensively investigated to potentially revolutionize biomaterials for bone regeneration.
Conclusion:
This review summarizes the chemical and biophysical properties of carbon nanostructures and discusses their functionality in bone tissue regeneration.
1. INTRODUCTION
A large clinical need exists for bone reconstruction, including underlying conditions and causes such as high-energy trauma (e.g. road traffic accidents), tissue degeneration (e.g. osteoporosis), infection (e.g. osteomyelitis), and developmental deformities [1]. In trauma and orthopaedic surgery, extended bone loss is associated with considerable technical and physiological issues. In the quest for alternative therapeutic strategies, research is focusing on the concept of tissue engineering to assist the progress of bone tissue regeneration.
Advantageous translation of tissue engineering strategies to several surgical specialties has motivated clinicians to adopt these approaches in bone tissue engineering of defects [2, 3]. The elemental approach underlying tissue engineering is to combine a biomaterial scaffold with cells, and/or biologically active cues to form a construct, which promotes regeneration of organs and tissues [4, 5]. The biomaterial scaffold design should consider physicochemical properties, microstructure, and degradation in vivo. A suitable scaffold will (i) possess an interconnected porous structure with surface properties that are beneficiary for the adherence, growth and differentiation of appropriate cells and enable the exchange of nutrients and metabolic waste, and (ii) be biodegradable with a rate to compliment tissue growth and regeneration [6].
Currently, there is also a need for better filler materials that can be used in the reconstruction of large bony defects and implants that are mechanically robust and suited to their biological environment. A material measuring less than 100 nanometers (nm) in at least one dimension is the widely accepted definition for a ‘nano’ particle, material or structure [7, 8]. Due to their unique properties, carbon nanostructures are being used for a variety of applications, including imaging, cancer therapy, drug delivery, and regenerative medicine [9, 10]. Carbon nanostructures have special mechanical, electrical magnetic, optical, chemical, and other biological properties because of their high aspect ratio and large surface area [11-14].
In this review we discuss the currently available reconstructive options for bony defects, followed by currently available biomaterials and tissue engineering techniques. Furthermore, we discuss the use of carbon nanostructures which enhance biomaterials in bone tissue engineering.
2. BONE RECONSTRUCTION
The traditional methods for management of bony defects include autografting and allografting cancellous bone, distraction osteogenesis, and applying vascularized free tissue transfer of the fibula and iliac crest [15, 16]. Despite several advantages, a number of drawbacks are associated with their use [17-20]. The generalized properties of bone reconstruction interventions have been summarized in Table 1 including their biophysical properties, the relative risk to the patient, and the practicability of the intervention (i.e. availability and cost) [17-31]. The biggest shortfall of allografts, xenografts, demineralized bone matrix (DBM), and alloplasts is that they lack either osteoinductive and/or osteoconductive properties, and in some cases lead to disease transmission and/or rejection [17, 19, 22].
The osteogenic, osteoinductive, and osteoconductive properties of autografts are ideal, whereas their use is associated with minimal risk of infectious disease transmission [19]. Since bone grafts are avascular and dependent on diffusion, the size of the defect and the viability of the host bed can limit their application. Autografts have restricted availability and are often difficult to harvest, causing additional morbidity. In addition, insufficient integration of bony autografts may necessitate secondary surgical procedures. Volume maintenance of the new bone can also be problematical due to unforeseeable bone resorption.
By definition, an allograft is tissue harvested from one individual and transplanted in another of the same species [32]. Use of bone allografts has similar drawbacks as autograft except the associated donor site morbidity, but increases the risk of infectious disease transmission. Fresh-frozen or freeze-dried allografts are available as structural and morselized forms [33]. Allografts are osteoconductive and provide a scaffold framework for host tissue to grow in. Contrarily, it has moderate osteoinductive properties at best. Following implantation, the host is believed to ellicit a complex immune response [33, 34]. Freeze-drying or freezing the allograft is imperative in restricting this immune reaction; although, the crucial osteoinductive, osteoconductive, and mechanical properties of the material may be reduced.
Vascularized bone transfers import vascularized tissue into the defect and can be used to bridge larger bone defects. They are, however, associated with significant donor site morbidity, limited availability, technical difficulties, and long operating times [35].
Distraction osteogenesis, a surgical procedure used to lengthen bone, entails a corticotomy to fracture the bone into two segments followed by distraction to allow osteogenesis in the gap. When distraction has led to the desired length, the bone is allowed to heal. This technique has the benefit of increasing the length of bone as well as the volume of the surrounding soft tissue. However, multiple procedures are required to achieve the desired results, considerable morbidity is caused to the patient, and there is a major risk of infection [36].
2.1. Biomaterials in Bone Tissue Engineering
Recent developments in the field of biomaterials and tissue engineering have led to many compounds and materials with a wide range of favourable properties to be used for implantation [37-39]. Oryan et al. have provided an excellent review on the classic options, as well as emerging biomaterials in bone tissue engineering; including a list of commercially available bone graft substitutes [17].
Bone Reconstruction Intervention |
Relative Properties | |||||||||||
---|---|---|---|---|---|---|---|---|---|---|---|---|
Biophysical Properties | Risk to Patient | Practicability | ||||||||||
Osteo- conductive |
Osteo- inductive |
Osteo- genic |
Osseo- integration |
Structural Framework |
Additional Morbidity |
Disease Transmission | Rejection | Availability | Cost | |||
Cancellous autograft [17, 19, 22] | +++ | ++/+++ | +++ | +++ | -/+ | ++/+++ | - | - | + | ++/+++ | ||
Cortical autograft [17, 19, 22] | ++/+++ | ++/+++ | ++/+++ | +++ | +++ | ++/+++ | - | - | +/++ | ++/+++ | ||
Distraction osteogenesis [23-26] | +/+++ | ++/+++ | ++/+++ | N/A | N/A | +/++ | - | N/A | + | ++/+++ | ||
Allograft [17, 19, 22] | +/+++ | -/+ | - | ++/+++ | -/+++ | - | + | +/++ | +/+++ | +/+++ | ||
Xenograft [17] | +/+++ | + | - | ++/+++ | -/+++ | - | ++ | +++ | ++/+++ | +/++ | ||
Deminieralized bone matrix [17, 19] | +/+++ | ++ | ++/+++ | ++/+++ | -/+++ | - | + | +/++ | ++/+++ | +/++ | ||
Alloplasts [17, 19] | +/+++ | -/++ | - | ++/+++ | -/++ | - | - | -/+ | +++ | + | ||
Polymers [27-29] | ++/+++ | ++/+++ | ++/+++ | ++/+++ | +/++ | - | - | - | +/+++ | +/+++ | ||
Carbon nanostructure composites [30, 40-43] | ++/+++ | ++/+++ | ++/+++ | ++/+++ | ++/+++ | - | - | - | +/+++ | ++/+++ | ||
Optimal intervention | +++ | +++ | +++ | +++ | +++ | - | - | - | +++ | + |
Bone with its complex molecular and anatomical components can be analysed as a ‘composite’ material. It consists of collagen, bone mineral, and water. The mineral component can be approximated as hydroxyapatite (HA) with the molecular formula: Ca10(PO4)6(OH)2), which in addition incorporates silicon, carbonate, and zinc. Some biomaterials currently used for bone regeneration aim to mimic its molecular microenvironment. Furthermore, the main function of bone is loadbearing and structural support. It has a high elastic Young’s Modulus ranging from 12 to 18 GPa [27].
To date, metals have been preferably selected as structural implants to replace or reinforce bone owing to their superior mechanical properties. The majority of the materials are, however, not suitable for implantation due to the host’s poor tolerance of dissolution products. Currently acceptable materials are based on titanium, cobalt, iron, nickel, zirconium, tantalum, gold and silver. Alloys have been made of these base metals aiming to improve their properties, including ultimate strength, elastic modulus, fabricability, ductile behaviour, and corrosion resistance. There are three alloy systems available: titanium alloys, stainless steel (chromium, iron, and molybdenum), and cobalt-chromium. These metallic implants, however, have many drawbacks including stress shielding, which may result in bone atrophy and osteoporosis, palpability, risk of infection, extrusion, and particulate leaching [45-47].
DBM is derived from allograft bone and prepared by pulverization of bone to a specific size. This process is followed by acid extraction of the mineralized part of bone [33, 34, 48], resulting in a mixture of collagen, growth factors, and proteins [48, 49]. In addition, different carriers, such as HA, glycerol, gelatin, and calcium sulfate can be added to the composite [48, 50]. DBM does not provide mechanical support, although its osteoconductive properties are highly desirable. It has superior osteoinductive properties compared to allograft bone due to the release of growth factors during the demineralization phase [33, 34].
Many other non-metallic materials have been investigated for implantation in bony defects, including bioglasses and ceramics, as well as polymers such as polymethylmathacrylate (PMMA) and ultrahigh molecular weight polyethylene (UHMW-PE). PMMA is mainly used to affix implants. Although adhesive, it does not bond to either the bone or the implant. UHMW-PE, applied as a biomaterial for orthopaedic implants, has a low coefficient of friction and a nonstick self-lubricating surface. The major disadvantage of UHMW-PE has been wear resulting in particle debris that is not biocompatible and can lead to loosening of the prosthesis. Ceramics have poor strength and are brittle. They have been mainly used for filling smaller bony defects. The most popular ceramics have been tri-calcium phosphate and the derived ceramic HA. Tricalcium phosphates are porous ceramics that mimic the microstructure of cancellous bone. Bioglass has a porous structure that supports tissue ingrowth and improves implant-tissue interface and stability. However, it’s more suited for load-free areas due to its low fracture resistance..
Synthetic bone substitutes, used as bone ‘fillers’, are vastly osteoconductive. They carry minimal risk of disease transmission and range from those with minimal osteoinductive capacity (calcium sulphate) to those with high osteoinductive capacity (Bone Morphogenic Proteins (BMP), such as BMP-2 and BMP-7). Calcium sulfate has been implanted as a bony substitute since the 19th century [33]. Current forms of processed calcium sulfate include Osteoset (Wright Medical, Arlington, TN, USA), and Bone Plast (Interpore Cross International, Irvine, CA, USA). Osteoset is indicated for filling small bony defects [51]. Coralline calcium phosphate has a highly organized microstructure, with large interconnected pores that simulate the structure of cancellous bone [51]. Pro-Osteon, a synthetized coralline calcium phosphate converted into hydroxyapatite by heat (Interpore Cross International, Irvine, CA, USA) is available for the treatment of metaphyseal defects [51]. It has a long resorption time, which is one of its initial disadvantages. Vitoss (Orthovita, Malvern, PA, USA) is a β-tri-calcium phosphate designated for bony defects of the pelvis, extremities, and spine [51]. Vitoss is excessively porous and promotes cell-mediated remodelling and resorption following implantation [51]. It, however, often requires the addition of bone marrow to function properly.
Both synthetic and natural polymers have been extensively investigated. Synthetic degradable polymers, such as polycaprolactone (PCL), polyvinyl alcohol (PVA), and polylactides (PLLA, PLDA), polyorthoester (POE), polyanhydrides, polyglycolide (PGA) and especially poly(lactide-co-glycolide) (PLGA) are most commonly used for bone regeneration [4].
Xenografts are transplantation from another species into humans, such as from bovine [52], porcine, and equine [53] sources. There are also many marine sources (such as coral, algae, cuttlefish, and others) which have also been identified to extract hydroxyapatite and/or calcium carbonate materials and scaffolds [54]. While potentially in high abundance, xenografts have low osteoinductive and osteogenic potential, as well as have the potential to cause cross-species rejection in some cases [55].
2.2. Advances in Materials for Bone Tissue Engineering
Lack of interactions or unsuitable interactions between synthetic materials and tissues remain a major concern; often resulting in implant failure [56-59]. When considering biomaterials suitable to support bone tissue engineering, these should stimulate and support both the in-growth of bone as well as remodelling and maturation of bone by providing geometrical cues and suitable stiffness. It is paramount that scaffolds provide sufficient initial mechanical strength to substitute for the loss of the diseased or absent tissue and biodegrade at a rate which is compatible with tissue ingrowth and maturation [4]. No single natural or synthetic compound has similar mechanical properties and bioactivity as bone tissue, which is made of both inorganic and organic components [60]. A common strategy to overcome this issue, and enhance implant biocompatibility has been to modify their surface and microarchitecture with functional moieties and to combine them with other materials to form composites [61-65]. A wide range of nano- and micro-scale materials have been utilized in bone tissue engineering, including natural and synthetic polymers [66, 67], ceramics [68-70], composites [27, 71, 72], metals [73, 74], and even extracts from coral [75, 76] or sponge, coming from either naturally-occurring (e.g. collagen) or synthetic (e.g. PLGA or PGA) sources [60]. Functional moieties are usually selected to generate biocompatible matrices by carrying specific cues and/or growth factors or non-fouling surfaces, preventing the adherence of undesired proteins and cells.
In addition to improving biocompatibility of implants and bone fillers, several strategies for improving the mechanical properties (compression and flexural) of polymeric scaffolds have been employed including that of synthetic biodegradable polymers. The integration of harder materials into polymeric scaffolds has been investigated for decades [77-79]. Noteworthy strategies include the addition of hydroxyapatite and tri-calcium phosphate [80]. The achieved improvement in mechanical properties from such strategies is still in the range of trabecular bone and not comparable to that of cortical bone. Majority of nonmetallic materials have not found widespread use as structural implants owing to inherent deficiencies in mechanical properties,. This has prompted scientists to design and develop mechanically compatible scaffolds that can accommodate the mechanical properties of bone [4, 81], are biocompatible, and induce bone regeneration.
3. CARBON NANOSTRUCTURE COMPOSITES
Over the last 20 years there has been a sharp rise in bone tissue engineering research [1, 4, 60, 66, 82], especially around the use of carbon nanostructures [30, 83-85]. Although carbon nanostructures are promising materials for bone tissue engineering for their light weight, strength, conductivity and stability [72, 86], they cannot be readily used in their pure form as synthetic bone tissue and have therefore been combined with other materials to create new composites [72, 86]. Consequently, carbon nanostructure-reinforced composites are an emerging class of high-performance materials with unique mechanical properties [41, 42, 64, 65, 72, 78, 87-95], having both a high Young’s modulus and tensile strength [27, 96-105] (Table 2).
Structure |
Tensile Strength (GPa) |
Young’s Modulus (GPa) |
References |
---|---|---|---|
Bone | |||
Cortical Bone | 0.051-0.133 | 12-18 | [27, 96, 106] |
Cancellous Bone | 0.0074 | 0.1-0.5 | [27, 106] |
Metal Alloys | |||
Stainless Steel | 0.586 | 190 | [106] |
Co-Cr alloy | 1.085 | 280 | [106] |
Ti-alloy | 0.965 | 116 | [106] |
Ceramics | |||
Alumina | 0.300 | 380 | [106] |
Zirconia | 0.820 | 220 | [106] |
Bioglass | 0.042 | 35 | [106] |
Hydroxyappetite | 0.050 | 95 | [106] |
Carbon Nanostructure | |||
Graphene | 130 | 1000 | [97, 98, 107] |
Graphene nanoribbons | 170-175 | ~1000 | [101, 108] |
Graphene oxide nanoplatelets | -- | 220 | [97, 99, 100] |
Carbon Nanotubes | |||
SWCNT | 126 | 650 - 5500 | [103, 104] |
DWCNT | 23-63 | -- | [116] |
MWCNT | >63 | 200 – 1950 | [103-105] |
Nanodiamonds | >60 | 170-1220 | [102, 103] |
3.1. Carbon Nanostructures
Graphene, a basic building-block of carbon nanostructures, is a honeycomb lattice of carbon atoms in a single layer. Graphene’s planar structure and sp2-hybridized carbon bonds enable it to react in a number of reactions, such as carbine insertion reactions, cyclo-additions, and click reactions [97]. It is one of the thinnest and strongest materials ever examined [107, 110]. Other carbon nano-scale allotropes include multi-walled carbon nanotubes (MWCNT), single-walled carbon nanotubes (SWCNT), buckyballs, ultra-short single-walled carbon nanotubes (US-SWCNT), graphene nanoribbons, and (graphite) nanoplatelets (Fig. 1), each with their own unique attributes.
Carbon nanotubes (CNTs) are cylinders of rolled-over sheets of graphene, with a typical diameter of a few nanometers and aspect ratios of up to 130 000 000:1. Typically, they are insoluble in water, but could be made soluble via chemical modification with single-stranded DNA or phospholipids containing a polyethylene glycol (PEG) moiety, for example. CNTs can be classified into two categories based on their structure: 1) single walled (SWCNTs), which consist of one layer of grapheme cylinder and 2) multi-walled (MWCNTs), which contain multiple layers of graphene cylinders [111]. SWNTs have photoluminescence properties that could be advantageous for applications in imaging and diagnostics, whilst MWNTs provide a larger surface, which could better facilitate external functionalization [112, 113].
Nanodiamonds (NDs), which are diamonds typically measuring 4-10 nanometres in diameter, are different from graphene-based materials as they are sp3 hybridized in a crystal lattice [114] rather than taking on planar architecture of graphene. NDs have excellent protein binding properties and can be carriers of molecules such as BMP-2 and basic fibroblast growth factor (b-FGF) [115]. Mochalin et al. provide a broad overview of ND synthesis, purification, de-aggregation, surface modifications, properties and applications [116].
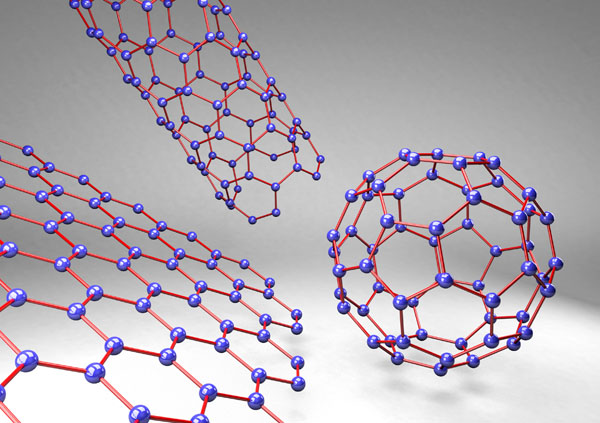
Pristine carbon nanostructures are incompatible with aqueous solvents and therefore much effort has been made to ‘functionalize’ these molecules. As such, carbon nanostructures, including graphene, CNTs and NDs, can be functionalized with alkyl, carboxyl, or other groups [117, 118] to improve solubility and dispersion [30]. These functionalizations can affect the carbon nanostructure’s physical properties, as well as its osteogenic, osteoconductive and osteoinductive abilities [6, 40, 71, 109, 117, 119-121].
3.2. Carbon Nanostructure-polymer Composites
The nano-dimensionality of carbon nanostructures offers potentially higher bonding energy and larger surface area. These properties increase the possibility of interfacing with the nanocomposite polymeric scaffold [79, 122]. Many biocompatible polymers, fillers, excipients, and other materials are currently being evaluated for clinical use, not only in bone tissue engineering. Seal [123], Rezwan et al. [27], Middleton et al. [28], and Armentano et al. [29] and others have reviewed some of the most common biodegradable, bioresorbable, bioerodable, and/or bioabsorbable polymers [4, 124], which could potentially be used in conjunction with carbon nanostructures as fillers. Other review articles and individual studies have investigated properties of carbon nanostructure-containing polymeric composites [64, 65, 71, 72, 87, 88, 125, 126]. While Mittal et al. [72] and Venkatesan et al. [30] summarize the properties of CNTs, graphene and polymers, many of the factors they describe can be applied to other carbon nanostructures and scaffolding materials. “Such properties not only depend on the inherent properties of the polymer and the filler, but also on the size and shape of the filler, the distribution of filler within the polymer matrix, and the physical and/or chemical interactions between the polymer and filler” [40].
Graphene and its planar derivatives [e.g. graphene oxide and reduced graphene oxide (nanoplatelets)] have been shown to be an effective filler in polymer composites, and in some instances, outperform CNTs as a filler [72, 127-129]. In some cases, such as with specific polymers, graphene may be the preferential carbon nanostructure of choice. For example, low nanofiller content graphene nanoplatelets performed significantly better than CNTs in terms of enhancing “tensile strength, Young’s modulus, fracture toughness, fracture energy, and resistance to fatigue crack growth” [128]. In another instance, MWCNTs were only shown to be effective in epoxy composites when ‘unzipped’ into graphene nanoplatelets, most likely due to increased surface area and the physical properties of epoxy scaffolding [129].
A wide range of literature reviews and studies exist summarizing the synthesis methods and physical properties of polymeric composites containing CNT fillers [43, 71, 72, 77, 79, 83, 85, 92, 109, 130-143]. Venkatesan et al. describe scaffolds from chitosan, collagen, gelatin, PCL, PLGA, and PLLA, among others [30]. Most of these composites show clear signs that CNTs enhance the polymers’ ability to replace bone tissue. The electrical conductivity of CNTs also brings an interesting element to the use of bone material, as electric stimulation of 10 mA at 10 Hz has shown to increase cell proliferation, increase extracellular calcium, and upregulate collogen type-1 [144].
Similar to graphene and CNTs, ND composites are also demonstrating effectiveness in bone tissue scaffolds [145, 146]. Mochoalin and Gogotsi have reviewed the literature for ND-polymer composites, which provides evidence that NDs can improve the properties of the composite, such as the “Young’s modulus, hardness, high thermal conductivity and electrical resistivity, low coefficient of friction, chemical stability, and biocompatibility” [146]. Of particular interest are octadecylamine modified ND (ND–ODA), and composites of poly(l-lactic acid) with ND–ODA, which are relatively non-toxic and have “Young’s modulus values close to cortical bone” [147]. NDs do not present the same high aspect ratios as CNTs, yet their spherical shape and large surface area per unit of particle volume allow NDs to have a few benefits over CNTs [146].
3.3. Carbon Nanostructure-ceramic Composites
Ceramics are of interest to bone tissue engineers due to their high stiffness, high strength, chemical inertness, and low density; but has a lack of toughness and are prone to catastrophic failures if defects exist [148]. The incorporation of carbon nanostructures aims to maintain the materials’ strengths, but also increases their toughness, prevents these catastrophic failures from occurring, and increases their functionality as bone tissue. Case in point, ceramics with functionalized graphene have improved physical properties and biological outcomes, such as enhanced osteogenicity (better adhesion of the hMSCs), calcium deposition by osteoblasts, sintering temperature, and electrical conductivity [149-151]. Simliarly, graphene nanosheet-HA nanorod (GNS-HA) composites display superior attributes to pristine graphene oxide or hyaluronic acid [152].
There are a range of studies which mix CNTs with ceramic(-like) materials [30] such as hydroxyapatite [153-160] and bioglass [69, 70, 151, 161-165]. CNTs significantly increase the “compressive strength and fracture toughness (by 106% and 21%, respectively)” [166] of diopside scaffolds at low concentrations (MWCNTs from 0.5-2 wt%). “Further, the scaffolds exhibited good apatite-formation ability and supported adhesion and proliferation of cells in vitro” [166].
3.4. Carbon Nanostructure-polymer-ceramic Composites
Increasingly, studies are evaluating the effects of polymer-ceramic composites [167-169], as well as with carbon nanotubes [80, 87, 162, 170-172]. There is still much work to be done as there are an almost limitless number of combinations of carbon nanostructures, polymers and ceramic(-like) compounds which could be mixed together. Early results do look promising. For example, 2-7% MWCNTs increased the roughness of a poly(3-hydroxybutryate)-bioactive glass composite surface, and in low concentrations, enhanced cell attachment and proliferation [162]. Additionally, Chitosan-MWNTs-HA nanocomposites demonstrated a sharp increase in the elastic modulus by 114% and compressive strength by 218%, by increasing MWNTs-chitosan weight ratios from 0 to 5% [90]. Plenty more examples of carbon nanostructures enhancing complex composites exist, yet the purpose of this review is not to list all previously evaluated formulations. A more thorough investigation into the literature is required, such as a systematic review of the formulations evaluated and the outcomes of such studies.
3.5. Effectiveness of Carbon Nanostructures in Bone Tissue
There are an almost an indefinite combination of carbon nanostructures, polymers, ceramics and other excipients which could be combined to create a potential material for bone tissue repair. Nonetheless, a few promising candidates have been tested both in the in vitro and in vivo settings. While there have been a large number of in vitro experiments evaluating carbon nanostructures, there have not been many which have evaluated carbon nanostructures for bone tissue engineering using in vivo models to date [9]. Nonetheless, carbon nanostructures continue to be some of the most promising materials for bone tissue engineering [9, 30].
Dubey et al. have recently summarized a wide range of studies evaluating’s graphene’s potential to enhance osteogenesis [173]. Newman et al‘s review thoroughly evaluates studies in the literature from 2002 to 2013 which evaluate the osteogenic and proliferative potential of SWCNT and MWCNT [119], as well as discusses the potentials and pitfalls of CNT design and use. Pristine MWCNTs have been shown to delay the growth of human bone marrow mesenchymal stem cell (hBMSC), however enhance differentiation. Cells “spread on MWCNTs toward a polygonal shape”, which is an important step in osteogenic differentiation [174]. In addition, hBMSCs preferred MWCNTs to tissue culture plastic for attachment and growth, supporting the osteoinductive properties of MWCNTs [174]. Nanodiamons are also biocompatible [91] and promote osteoblast adhesion and proliferation (osteoconduction and osteogenesis) [175].
SWCNTs and MWCNTs have been shown to be used alone as scaffolding to promote bone cell (osteocytes and osteoblasts) proliferation in vitro [176]. A wide range of studies have shown positive effects of CNT on bone cell proliferation [86, 159, 177-183]. In 2002, Supronowicz et al. demonstrated for one of the first times that CNT composites can promote osteoblast functions that are responsible for the organic and inorganic phases of bone composition [144]. Since then, several carbon nanostructure composites, such as carbon nanofiber-polycarbonate urethane (PCU), CNT-poly(lactic acid) (PLA) and CNT-PLGA, were shown to enhance adhesion of osteoblasts in vitro [83, 184-186].
Usui et al. have exhibited that MWCNTs implanted into ccy mice “show high bone-tissue compatibility, permit bone repair, become integrated into new bone, and accelerate bone formation stimulated by recombinant human bone morphogenetic protein-2 (rhBMP-2)” [31]. Bhattacharya found that when SWCNT composites were placed in a critical-sized rat calvarial defects, there were few signs of rejection or inflammation and showed adequate bone formation was [187]. Similarly, carbon nanohorns, a type of CNT, have been shown to be both compatible and effective in bone tissue regeneration within rat calvarial bone defects [188]. Likewise, nanodiamonds have also been promising materials in vivo. Addition of oxygen-terminated ND particles to a poly(L-lactide)-co-(e-caprolactone) scaffold significantly increased bone marrow-derived stem cell attachment and proliferation in vivo, along with enhanced bone formation after 24 weeks in vivo [189], which has also been confirmed in vitro [190].
4. TOXICITY OF CARBON NANOSTRUCTURES
While carbon nanostructures have been described as “extremely effective and very safe biomaterials” [9] in both in vitro and in vivo testing for bone engineering applications [191], carbon nanostructures present toxicological effects [191-201]. This toxicity should be taken into account when engineering synthetic bone tissues. It must also be noted the release of CNTs can occur not only during production, but also during the usage and disposal phases of these applications [202].
The pharmacodynamics, pharmacokinetics, and toxicokinetics of a carbon nanostructure can vary significantly based upon the specific dimensions, surface properties/functionalization, bio-durability and the protein corona surrounding the carbon nanostructure [193, 203]. As the toxicological effects of a carbon nanostructure will most likely differ once combined into a composite, and the fact that many composite materials have been independently tested for safety, we have focused on describing the toxicological effects of common carbon nanostructures below without potential composite materials. Complicating the matter is the fact that studies are difficult to compare due to there being different species, dosing, vehicles, functionalizations, and characterisation techniques; resulting in problematic interpretation and inadequate risk-assessment [192, 204].
4.1. Biodistribution and Biodurability
Biodistribution and biodurability of carbon nanostructures is of key concern when developing implants containing these materials. Until this process is understood, their use in the clinic will be obstructed [205]. Pristine CNTs have been found both at the site of exposure as well as other tissues up to 24 months post-administration [206-209]. The biodistribution of carbon nanostructures is primarily based upon the allotrope and its chemical functionalization. Pristine (non-functionalized) carbon nanostructures appear to be of most concern in regard to in vivo accumulation. Following intravenous injection, “pristine SWCNTs distributed the entire body with major accumulations in the liver, lungs, and spleen over an extended period of time” [210]. Similarly, when pristine MWCNTs were injected into mice, they “accumulated in the liver, lungs and spleen” [211].
Functionalizations can dramatically change a CNTs pharmacokinetic properties and biodegradability [212]. One study finds that the accumulation of CNTs is ameliorated by the degree of functionalization [213] rather than the functionalization’s characteristics (e.g. chemistry or size) [211], however other studies find that degradation rates are functionalization-specific [214]. Ali-Boucetta and Kostarelos suggest functionalization leads to rapid excretion through the bladder-urine route, whereas non-covalently coated CNTs show major accumulation in the liver and spleen [205].
4.2. Cytotoxicity
Cytotoxicity of carbon nanostructures has been observed along a range of cell lines, concentrations, production methods, excipients and assays [215]. However, recent studies have shown that CNTs have relatively low levels of cytotoxicity and are safe for clinical use [9, 185].
Studies suggest that there are two main mechanisms of carbon nanostructure toxicity: inflammation and reactive oxidative species (ROS), which are inter-related [192, 216]. Production of ROS results in lipid peroxidation, protein alteration, and intracellular GSH depletion, leading to NF-kB activation which in turn upregulates IL-1β, IL-6, TNF-α, iNOS (proinflammatory markers) to trigger cell death [216, 217].
Carbon nanostructures have some properties which make them cytotoxic, particularly to immune cells. Carbon nanotubes (CNTs) and fullerenes have been shown to by cytotoxic to alveolar macrophages [199], as well as induce human T-lymphocyte [218] and fibroblast [198] apoptosis in culture. Surface oxidation of CNTs has demonstrated toxicity in vitro, inducing apoptosis in human T lymphocytes [218]. Surface oxidation of CNTs by acidic treatment leads to a dose-dependent cytotoxicity to human neuroblastoma cells [219]. In a mouse model, MWCNTs coated with an acid-based polymer led to higher levels of macrophage-induced inflammation and oxidation [220].
The dimensions, such as length, width, and aspect ratio of a carbon nanostructure can potentially determine its toxicological properties. Murphy et al. demonstrated that for CNTs there is a length-dependant increase in acute cytokine release from THP-1 macrophages, which is most likely due to frustrated phagocytosis [221]. There are conflicting results on whether thinner CNTs appear to be more toxic than thicker CNTs [193, 195, 196, 222], however CNTs with lower aspect ratios demonstrate greater toxicity to human lung-tumor cell lines [223]. Imperfections or defects in carbon nanostructures can also play a role in undesired toxicity. MWCNTs present higher levels of genotoxicity with higher levels of defects [224].
Carboxyl groups have be implicated as a pro-inflammatory functionalization on CNTs [193, 225]. Lanone et al. suggest toxicity could be due to increased bioavailability, negative charge of the functionalization (possibly leading to higher levels of internalisation by macrophages), or both [193]. Conversely, amino [226] and polyethylene glycol (PEG) [216] groups can reduce CNT cytotoxicity, whereas albumin coating can specifically reduce SWCNT toxicity [227]. Furthermore, Wang et al. demonstrated that Pluronic F108 coating decreases (while albumin increases) the lung fibrosis potential of MWCNTs by reducing lysosomal injury [228], suggesting that it is important to consider the excipient used with carbon nanostructures in a composite.
Some experiments have shown that nanodiamonds have little to no cytotoxicity or genotoxicity across a range of tissues [229]. However other studies have detected ND-mediated cytotoxicity, proliferation, inhibition and oxidative stress in HeLa cells, as well as genotoxicity in higher concentrations [230]. Further evaluations are necessary to fully assess the toxicology of nanodiamonds.
4.3. In Vivo Toxicity
Acute and sub-chronic mouse and rat studies are summarized by Clichici et al. [192], Lanone [193] and Zhao et al. [215]. They all recommend standardized testing of carbon nanostructures due to the complicated nature of the molecule class and the lack of consistent testing in the literature. Similar to the cytotoxic effects of carbon nanostructures, ROS and inflammation appear to be the main causes of toxicity, leading to inflammation, fibrosis, granulomas and apoptosis [215]. Following implantation of CNTs, a majority of in vivo studies have reported on local reactions [9].
A wide range of inhalation toxicology studies have been performed evaluating CNTs as it is believed that CNTs are generated in the combustion of fuels [231-233] as well being an occupational hazard during CNT manufacturing [191, 234]. Lanone et al. [193] describe how the size and dimensions of a (carbon nano-) ‘particle’ or ‘fibre’ effects how a nanostructure might react in the lung. Gernand and Casman’s meta-analysis of carbon nanotube pulmonary toxicity studies [235] found that the metallic impurities, CNT length and diameter, and aggregate size contribute the most to pulmonary toxicity. A nanoparticle may be more confined to the lungs whereas a nanofibre would have a greater effect on the pleura (e.g. like asbestos). It has been shown in two different mouse models that long MWCNTs “results in asbestos-like, length-dependent, pathogenic behaviour” [236, 237]. Following intrapharyngeal or intratracheal administration of SWCNTs and MWCNTs in mice and rats; “inflammation, epithelioid granulomas (microscopic nodules), fibrosis, and biochemical/toxicological changes in the lungs” [191] were observed, independent of how they were synthesized, or the metal content or type [191, 200, 201]. Furthermore, in a comparative inhalation study in mice, “SWCNTs were more toxic than quartz” [191], a common reference material and known chronic occupational health hazard [200, 201]. Although inhalation toxicology may pose a threat to those manufacturing the CNTs or other carbon nanostructures, evidence suggests there should be minimal inhalation exposure while a clinician installs a carbon nanostructure-based composite implant [238].
Further research, methods development and standardization are required to truly evaluate a carbon nanostructure composite’s toxicological profile over its life cycle [238], especially once implanted in a patient.
4.4. Human Exposure Toxicity
Although they are promising materials, carbon nanostructures for bone tissue engineering are currently in the preclinical stage of development. Huckzo and Lange have evaluated fullerene soot with a high level of SWCNTs was tested to asses topical activity in human volunteers, which resulted in no signs of skin irritation or allergic risk [239]. However, as of October 2015, the authors have not found an evidence of carbon nanostructures being used in randomized clinical trials registered in European Union (clinicaltrialsregister.eu) or United States (clinicaltrials.gov). It is also unclear when these technologies might enter clinical trials for therapeutic purposes.
CONCLUSION
Carbon nanostructures present an interesting case for bone tissue engineering. They can be used as an additive to currently available materials to form a composite which is osteogenic, osteoconductive and osteoinductive. It is clear that there are a wide range of studies which paint an incomplete, yet informative, picture regarding carbon nanotube functionality and toxicity as a potential filler for bone tissue engineering [192, 205]. Ideally, a composite, whether made from carbon nanostructure(s), polymer(s), and/or ceramic(s), would have to possess all of the biophysicochemical and biomedical properties required of a bone substitute, without exorbitantly increasing the cost to manufacture the goods. Once a composite containing carbon nanostructures is refined and selected, it will require thorough investigations both in vitro and in vivo to ensure efficacy and safety as required by the regulatory agencies. If developing a new composite for clinical application, it would be wise to ensure this formulation was differentiated enough from the intellectual property landscape to allow commercial development of a product through the cost and time required to undertake and complete clinical trials.
While some allotropes of carbon present cytotoxicity and inhalation toxicity in certain instances, carbon nanostructures appear to be safe enough to continue to be evaluated for clinical use. There is evidence that shows that once a carbon nanostructure is fixed into a solid composite form, the effects of carbon nanostructure toxicity can be reduced [90], possibly due to reduced bioavailability, both in particulate form and/or in solution. Toxicokinetic studies suggest that a high level of functionalization of carbon nanostructures might be preferential in vivo. Once a carbon nanostructure detaches from an implant, this high degree of functionalization could prevent bioaccumulation in the liver and spleen. In the case of a long-term implant for bone defects, persistence at 24 months may or may not be beneficial, depending on the level of resorption desired. Based on this potential stability, it may be worthwhile to consider carbon nanocomposites for other uses than only bone defects, such as for joint replacement or full prosthetics.
Currently, the literature is fractured with pockets of combinations for fillers and scaffolding molecules being tested. What is missing from the literature is a systematic evaluation (e.g. factorial experiment/design of experiment) of different carbon nanostructures, polymers, ceramics, cements, bioglasses, fillers and/or excipients in a single evaluation platform. Furthermore, standardized experimental models and desired outcomes (e.g. Young’s modulus, elastic strength, HA deposits, etc.) to evaluate engineered bone tissue have not yet reached consensus in the academic community.
Although carbon nanostructures show promise, it must be noted that the high cost of carbon nanostructures has been an obstacle to widespread adoption [21]. The high cost of carbon nanostructures becomes even more exacerbated within regulated biomedical applications, as the cost of cGMP-grade material could be further prohibitive. Just as with DNA sequencing, demand and production of carbon nanostructures will increase. With economies of scale, production costs of carbon nanostructures should (hopefully) reduce significantly to allow further testing and evaluation.
Lastly, other technologies, such as cellular therapies, platelet therapies, growth factors, and other regenerative medicines are being developed in parallel to carbon nanostructures. It is unclear which of the technologies might be the most effective or safe, but it is clear that there is potential for these technologies to be combined synergistically [10, 76, 240-242].
LIST OF ABBREVIATIONS
b-FGF | = Basic fibroblast growth factor |
BMP | = Bone morphogenic protein |
cGMP | = Current good manufacturing practice |
CNT | = Carbon nanotubes |
Co | = Cobalt |
Cr | = Chromium |
DBM | = Demineralized bone matrix |
DNA | = Deoxyribonucleic acid |
DWCNT | = Double-walled carbon nanotubes |
GNS-HA | = Graphene nanosheet-hydroxyapatite |
GPa | = Gigapascals |
GSH | = Glutathione |
HA | = Hydroxyapatite |
hBMSC | = Human bone marrow mesenchymal stem cell |
MWCNT | = Multi-walled carbon nanotube |
ND | = Nanodiamond |
ND–ODA | = Octadecylamine modified nanodiamonds |
nm | = Nanometers |
PCL | = Polycaprolactone |
PGA | = Polyglycolide |
PLDA | = Poly-D-lactide |
PLGA | = Poly(lactide-co-glycolide) |
PLLA | = Poly-L-lactide |
PMMA | = Polymethylmathacrylate |
POE | = Polyorthoester |
PVA | = Polyvinyl alcohol |
ROS | = Reactive oxidative species |
SWCNT | = Single-walled carbon nanotube |
Ti | = Titanium |
UHMWPE | = Ultra-high molecular weight polyethylene |
US-SWCNT | = Ultra-short single-walled carbon nanotube |
CONFLICT OF INTEREST
The authors confirm that this article content has no conflict of interest.
ACKNOWLEDGEMENTS
We would like to thank Steve Atherton MA RMIP MIMI from the medical illustration department of Aberawe Bro Morgannwg University Health Board for generating (Fig. 1).