All published articles of this journal are available on ScienceDirect.
Nanoscale Surface Modifications of Medical Implants for Cartilage Tissue Repair and Regeneration
Abstract
Background:
Natural cartilage regeneration is limited after trauma or degenerative processes. Due to the clinical challenge of reconstruction of articular cartilage, research into developing biomaterials to support cartilage regeneration have evolved. The structural architecture of composition of the cartilage extracellular matrix (ECM) is vital in guiding cell adhesion, migration and formation of cartilage. Current technologies have tried to mimic the cell’s nanoscale microenvironment to improve implants to improve cartilage tissue repair.
Methods:
This review evaluates nanoscale techniques used to modify the implant surface for cartilage regeneration.
Results:
The surface of biomaterial is a vital parameter to guide cell adhesion and consequently allow for the formation of ECM and allow for tissue repair. By providing nanosized cues on the surface in the form of a nanotopography or nanosized molecules, allows for better control of cell behaviour and regeneration of cartilage. Chemical, physical and lithography techniques have all been explored for modifying the nanoscale surface of implants to promote chondrocyte adhesion and ECM formation.
Conclusion:
Future studies are needed to further establish the optimal nanoscale modification of implants for cartilage tissue regeneration.
INTRODUCTION
Articular cartilage damage, caused by degenerative and traumatic joint disease is a very common pathology causing pain and disability for patients [1]. Natural cartilage repair is ineffective due to the low self-healing abilities of articular cartilage [1]. There are several procedures available to repair focal cartilage damage [1]. Current clinical treatment options include non-implant based procedures such as microfractures and chondroplasty with the aim to smooth the damaged cartilage [1]. Alternatively, grafting procedures can be performed where autografts or allografts of articular repair from donor sites can be inserted into the damaged site [1]. Other techniques include cell-based techniques, which include autologous chondrocyte implantation (ACI), where chondrocytes are taken from the patient expanded in vitro and then re-implanted at the defect site. Alternatively, synthetic materials can be used to bridge the gap, which can degrade with time and allow new cartilage to regenerate or act as non-biodegradable implants and stay there for the lifetime of the patient. To date, it is not clear which method is the most effective [2]. Similarly, facial cartilage defects including nasal cartilage are also pathologies caused by trauma, degeneration but also congenital deformities and cancer resections [3, 4]. Craniofacial surgeons currently utilize autologous cartilage from elsewhere in the body to carve cartilaginous nasal replacements to restore the defect site. However, this is limited by donor site morbidity, pain and limited accessibility of suitable cartilage [3, 4]. Synthetic replacements to restore cartilage defects are available but with their high extrusion and infection rates, means that they are not suitable alternatives [3, 4].
A plethora of materials have been investigated for cartilage tissue engineering including synthetic materials such as polymers, ceramics, metals and composites of these [5, 6]. Furthermore, natural materials such as collagen, chitosan and gelatin have been used alone or in combination with synthetic materials [7-10]. When designing an implant for cartilage regeneration there are several factors to consider [11]. The characteristics of the implant surface are one of these extremely important parameters. The implant surface is critical for cell adhesion, which will consequently allow cell differentiation and tissue regeneration. Several factors influence the interaction of the cells with the implant surface including the roughness (topography), wettability (water loving ability) and chemistry. Fabrication of a patterned surface has shown to be a promising tool in tissue engineering and for the generation of cartilage [12].
Nanotechnology is defined as the engineering used in designing and creating structures that have at least one dimension that is on the nanoscale. In practice for medical devices, this can mean the implant contains a material that is at the nanosize (has one dimension that ranging from 1 and 100 nm) or has a surface that contains a nanotopography. Cartilage is hierarchical structure that consists of extracellular components of a complex network of pores fibres and ridges that are of the nanometer sized dimensions [13]. This structure has the precise architecture and topographical cues to support cartilage formation by directing cell adhesion, migration and differentiation [14]. Therefore, it is not surprising that research is exploring different approaches to mimic this nanoenvironment using scaffolds architecture, to successfully regenerate cartilage. Creating nanoscale surfaces on materials has shown the ability to be able to direct cell behaviour and directly control tissue formation.
In this review, different techniques for modifying implants to create nanosurfaces for cartilage regeneration will be discussed including simple methods such as physical and chemical modifications and the more complex lithography techniques. The advantages and disadvantages of each technique will be discussed in addition to providing examples of their success for the regeneration of cartilage.
THE IMPORTANCE OF NANOSCALE GEOMETRY
To reproduce a complex and functional tissue such as cartilage it is important to provide a biomimetic microenvironment that includes the biochemical cues and also the nano-topographical features for the cell to interact with the matrix [15]. There is a specific sequence of events that take place, when implants are in contact with body fluids. Firstly, protein adsorption onto the implant (plasma fibronectin) occurs, which later provides the important anchor sites for cell attachment. Nanoscale topography is now an established method of altering protein adsorption on implant surfaces. The wettability, surface energy and topography of the implants surface all alter protein adsorption. Several studies have shown that increased protein adsorption on nanomodified surfaces compared to smooth surfaces [15, 16].
After the protein has been adsorbed to the surface, the cells will attach to the surface, which takes place rapidly [15]. Cell adhesion takes longer and is dependent on the cytoskeletal, matrix and membrane protein arrangement of the cells. The cell spreading and migration will then take place, which again is dependent on the cell’s matrix and membrane proteins status [16].
Cells adhere to the surface at distinct contact points, called focal contact, close contact and extracellular matrix contacts [16]. Focal contacts present specific receptor proteins called integrins, which are responsible for the cell-substrate connection [16]. The adhesion of cells to the substrate surface through these integrin receptors is highly important in maintaining the anchoring of the cells and triggers signals that later direct the cell proliferation and differentiation [16]. The integrin receptors are the interface between the intra (cytoskeleton) and extracellular (matrix proteins) space. The surface density of specific receptor ligand bonds is important in cell adhesion. The density and spacing of ligands has shown to influence cell-adhesion [16]. The RGD sequence is a very important mediator to control cell adhesion onto surfaces [16]. The surface density of RGD peptides has shown to cause changes in cell adhesion and a minimum density has shown to be critical for the occurrence of cell adhesion. Cell adhesion has shown to be dramatically reduced when the ligand spacing is greater than 67 nm [16]. Other proteins like talin, paxillin and vinculin are responsible for the interaction between the actin and membrane receptor proteins. These proteins are important for cell attachment and for signal transduction [16]. Therefore, as cellular adhesion is vital to the long-term function of the cell including migration, proliferation and differentiation, research has explored techniques that optimise the implant surface for cell adhesion (Fig. 1) [15, 16].
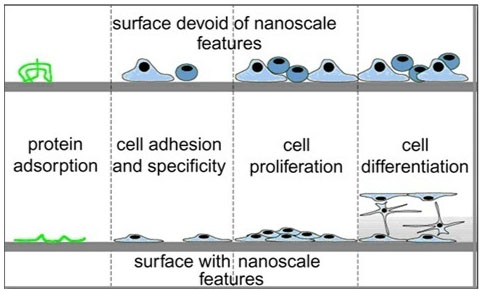
As cells in vivo respond to nanotopographical cues from their extracellular matrix (ECM) to guide cell adhesion, recent interest as been to recreate this nanoscale dimension on the surface of implants [17]. Significant work is being undertaken to understand and observe the behaviour of cells in the presence of nanotopography. Groove and pillars are the most common topography investigated when analysing the effect of topography on the behaviour of cells [17]. Furthermore the spacing of the topographies has been shown to influence cell behaviour. For example, 5 and 10 um pillars spacing caused differences in the arrangement of the cytoskeleton and migration in vitro [17]. Cell shape has shown to be affected by the underlying topography [17]. Filopodia and lamellipodia have shown to extend parallel to the nanoridge feature with alignment of their stress fibers [17]. Cell behaviour has shown to be influenced on the surrounding environment including the proliferation and differentiation. For example, human cell lines grown on different nanoarchitectures including randomized nanoscale bumps or nanoislands of varied heights <100 nm [17]. Cells were shown to maintain lower proliferation rates on larger nanoscale features [17]. Nanotopography has shown to control cell differentiation, for instance human mesenchymal stem cells have shown the osteogenic differentiation on nanopits and nanotubes in the absence of osteogenic induction medium [17].
METHODS OF IMPARTING NANO-FEATURES
Until recently, research was restricted to creating microtopographies to influence cartilage engineering due to the cost of creating nanotopographies. However recent progress in material process techniques has allowed the creation of nanotopography surfaces to become more feasible. There are various methods to generate nanoscale features at the implant surface, or a term called ‘nanopatterning’. These methods include i) Physical Methods ii) Chemical methods iii) Lithography and contact printing as shown in Table 1.
CHEMICAL METHODS
There are several chemical methods used to modify implants to create surface that can be used for tissue regeneration. Titanium surfaces can be modified to have nanotubular structures using anodic oxidation [18]. This process involves an electrochemical method for the production of an oxide film on a metallic surface [18]. The treatment is often used on metals to increase the corrosion resistance and wear resistance. Anodized metal including titanium have shown to promote chondrocyte adhesion and migration [18]. Although this study has only been conducted at the in vitro levels and further evidence is required to understand the true potential of regenerating cartilage in vivo using anodized metal [18].
Technology | Method | Advantages | Disadvantages |
---|---|---|---|
Nanoimprinting | Family of techniques, which use elastomeric stamps or mouds to transfer patterns to subtrates at the nanoscale. | High resolution (5-10 nm) High throughout Low cost |
High temperatures required for thermal based systems and difficulty in transfer pattern using UV systems. |
Microcontact printing | Patterns substrates by applying self assembled monolayers on an elastomeric stamp | Simple Low cost Versatile |
Lower resolution and reduced reproducibility (35 nm) |
Replica moulding (REM) | Use PDMS mould to cast the pre-polymer, which after the polymer is cured is detached. | Good resolution (30nm) Versatile Low cost |
Limited use of moulds |
Acid treatment of metals is often used to create nanosurfaces to improve their biocompatibility [19]. Acids commonly used are hydrochloric acid, sulphuric acid and nitric acid. These acids produce a uniform and clean implant, by removing contamination and creating an oxide layer [19]. As a result of the treatment there is a roughness, which increases the surface areas of the implant. Alkali treatment is a similar treatment, which involves immersing metals in sodium or potassium hydroxide at 800 degrees for 20 minutes [19]. This is often used for metal implants creating a nanostructure surface and bioactive sodium titanate layer, which has shown to be bioactive when placed within the body fluid. The titanate layer has shown to be a bioactive surface acting as a site for nucleation of calcium phosphate, which is useful for bone tissue engineering [20]. Sodium hydroxide of titanium has shown to be an affective method to promote cartilage adhesion. Kay et al. demonstrated that chemically treated titanium with sodium hydroxide (NaOH) promoted the adhesion of chondrocytes and osteoblasts due to the production of nanometer polymer surface roughness dimensions [21]. Hydrogen peroxide can cause oxidation of metal implants resulting in a nanostructured surface. Titanium has shown to create a titanium peroxy gel when in contact with hydrogen peroxide. Although acid and alkali methods have shown to promote the chondrocyte adhesion and growth there are drawback to these techniques. There must be great effort into washing the substrates after the treatments including acidic and basic treatment to ensure the pH does not effect cell viability [22].
Sol-gel method is a common technique used to deposit calcium phosphate (CaP), titanium dioxide (Ti-O2)-CaP composites and silica-based gels on surface materials [23]. The sol that forms is a group of submicroscopic oxide particles in liquid [23-25]. The gel that forms on the implant surface is dependent on the roughness, chemical state and temperature used in the process. Mohan et al. investigated the potential of electrospun fiber assembled hydrogel with gradients of chondroitin sulphate (CS) and sol-gel derived bioactive glass (BG) to create cartilage in three-dimension [24]. The fibers were able to support the chondrocytes to secrete hyaline like matrix with high levels of sulphated glycosaminoglycans (sGAG), collagen type II and aggrecan. Demineralised bone matric (DBM) a chitosan hydrogel and a BMSC-specific affinity peptide was used for in vivo cartilage formation [25]. The biphasic scaffold platform retained more cells homogenously within the sol-gel transition of chitosan [24]. Six months after in vivo implantation the biomaterial showed superior cartilage without complications compared with routine surgery as demonstrated by MRI and histology [24].
Chemical vapour deposition is another technique that can deposit compounds on the surface due to a chemical reaction-taking place between the implant surface and chemicals in a gas phase [26].
PHYSICAL METHODS
There are several physical methods, which can engineer nanosurfaces that are less than 100 nm in dimension that have been used for cartilage regeneration [27]. Plasma spraying is a highly investigated technique that has been implemented to improve the wettability of the surface by altering the surface chemistry and roughness of a surface to improve cell adhesion [28]. This technique can be utilised for many different materials types including polymers, ceramics, and metals [28]. Plasma treatment involved bombarding the surface with highly excited atomic, ionic and radial species. These are obtained when gases are excited into an energetic state by either radiofrequency, microware or electrons discharged from a hot filament [28]. By choosing an appropriate plasma source, functional groups can be introduced onto the implant surface changing the surface chemistry and wettability, which can affect cellular behaviour including their adhesion, proliferation and differentiation [28]. Plasma surface modification has shown to improve the surface for chondrocyte attachment and also support the chondrogenic differentiation of MSCs [29]. Surface modification of electrospun PLLA nanofibres by plasma treatment and immobilisation of gelatin demonstrated an ideal scaffold materials for cartilage tissue engineering. Electrospun poly(lactic acid) (PLLA) nanofibers (NF) were treated with oxygen treatment to introduce -COOH (CG) groups on the surface, which was followed by the covalent grafting of CG molecules onto the fiber surface, using water-soluble carbodiimide as the coupling agent [29]. Chondrocytes shown that they were able to maintain their expression of chondrocyte markers including collagen II, aggregan and SOX-9 [29]. Subcutaneous implantation of the cell scaffold also allowed the formation of ectopic cartilage tissue after 28 days in vivo [29]. To create an ideal biomimetic tissue scaffold for stimulate cartilage regeneration with functional properties, Zhu et al. used cold atmospheric plasma (CAP) treatment with sustained growth factor delivery via microspheres [30]. The CAP technique is not like traditional high temperature plasma but is an ionized gas whose temperature is close to room temperature composed of a unique environment of charged particles [30]. The CAP treatment improved the hydrophilicity of the scaffolds. Furthermore, CAP and microspheres can act synergistically and enhance the differentiation of human mesenchymal stem cells (hMSCs) as shown by the increase in glycosaminoglycan, type II collagen, and total collagen production [30]. The CAP also enhanced the 3D cell infiltration into the scaffolds. Balasundaram et al. evaluated highly porous nanoscale roughness on polyurethane and polyurethane (PU) and polycaprolactone (PCL) [22]. Nanoembossed polycarbonate urea (PCU) and PCL were created by casting of PU and PCL over a plasma-deposited, spiky nanofeatured crystalline titanium (Ti) surface [22]. There was a greater roughness, higher surface energy as compared to unmodified polymer resulting in greater chondocycte adhesion and protein production. The authors concluded that nanomodification of PCU and PCL improved cartilage function and thus deserved further investigation [22].
Sputtering is another technique, which can be utilised to eject atoms or molecules by the bombardment of high-energy ions creating bio ceramic thin films [31]. This technique has been used to improve the wear and corrosion of metals [31]. Ion implantation is another specialised technique, which causes the atomic rearrangement of the substrate. The process involves ions of the desired element to be produced at the ion source and accelerated in an electrical field and then impacted onto the material [32]. Ion implantation has been used on metals to prevent the chemical correction and wear due to friction [32]. To date there is a lack of evidence for the utilisation of sputtering and ion implantation technique to create suitable scaffolds for cartilage tissue engineering.
A highly advanced technique involves laser technology, which can produce structures on the surface to the nano and micro level [33]. The implants surfaces created using laser have high resolution, creating precise and targeted surface patterns [33]. Furthermore, the process is very rapid and clean [33]. However, there has been a lack of research into creating nanoscale patterns for cartilage tissue engineering.
LITHOGRAPHY
Whiteside and colleagues developed a technique to pattern surfaces called ‘soft lithography’ in 1997. These non-photolithographic techniques broadly utilise three main techniques, which are all based on using an elastomeric stamp to mould desirable two-three 3D micro and nanoscale patterns on different substrates [34, 35]. Soft lithography is a group of techniques which can be broadly broken down into three groups i) printing ii) replica moulding and iii) embossing.
Printing is defined as process that involves material transfer from the mould onto the substrate and can be either microcontact printing or nanotransfer printing [35]. Microcontact printing is a lithographic method, which is based on an inking approach and has become widely used to create functional surfaces for tissue regeneration [35]. The process involves using an elastomeric sitemap with self assembled monolayers, which act as an ink [35]. The self-assembled monolayers are then transferred to the substrate creating the required pattern [35]. For tissue engineering microprinting should be seen as a complementary method to pattern surfaces by creating chemical cues and can be used with other techniques that create topographies [35]. This technique is simple, cheap and versatile with the capability of printing 500 nm structures [35]. However, there may be stamp demonstration after removing the template and has a lower resolution compared to other techniques. Pan et al. demonstrated the influence of collagen IV patterns on chondrocyte behaviour using microcontact pattering on polystyrene strips [36]. Polydimethylsiloxane (PDMS) stamps were prepared using optical photolithography and then the collagen deposited by microcontact printing. It was clear that the chondrocytes preferred to attach to the protein area and that the cell shape was different depending on the width and spacing of the protein [36]. Microprinting is useful to understand the guidance cues for the control of chondrocyte adhesion and cell morphologies [36].
Replica moulding (REM) is a process, which uses a soft mould and a photo or thermal curable pre-polymer [35]. The pre-polymer is cast into a PDMS mould and then solvents are photo or thermally removed to produce the micro/nano structures on the polymer substrate (Fig. 2) [35]. Replica moulding (REM) is useful as it is possible to make patterns on non-planar rigid and soft substrates at a reasonable cost [35]. However, some of the pre-polymers dissolve the PDMS mould so there is a maximum number of times the mould can be utilised. Ragetly et al. utilised replica mould printing for cartilage tissue engineering using chitosan scaffolds [37]. Fibrous scaffolds of different sizes were produced used replica moulding technique. Porcine chondrocytes maintained their phenotypic appearance and viability on the chitosan scaffolds [37].
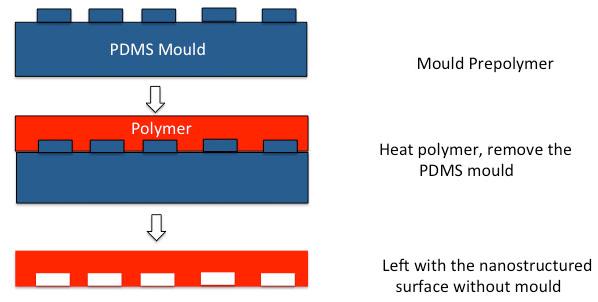
Hot embossing is another technique that can be used to create patterns of different topographies on surface for tissue regeneration [35]. This is a process that involves temperature pressure and vacuum in order to mould polymer features as low as 5 nm onto the substrates surfaces to guide cell responses [35]. Hot embossing can be termed ‘nanoimprint lithography (NIL)’ when creating patterns as the nanoscale as the master mould are silicon wafers to create the high resolution and ‘embossing’ or ‘micro hot embossing’ when the structures are in the order of μm [35]. The NIL technique offers many advantages over optical lithographical technique that depends on electron beam scattering as NIL causes a uniform mechanical deformation of the substrate [35]. In NIL mechanical embossing is applied on the resist that then serves as the replica of the original pattern [35]. The NIL technique provides high-resolution scaffolds but is associated with various etching processes, which increase the time and costs and therefore it is not a commercial process (Fig. 3) [35]. In thermal NIL, a fine layer of thermoplastic polymer is deposited on a silicone wafer, which is then spin coated to form the surface of the imprint resist [35]. A hard mould with the desired nanostructure is then embossed into the resist surface by a thermo-mechanical process, this ensures the desired nanostructure of the mould are in the polymer resist. Alternatively ultraviolet (UV)-NIL, is created by coating the substrate surface with a UV curable liquid resist [35]. The mould is then pushed into the substrate with the nanotopography before UV radiation is applied to solidly the resist [35]. This process is lower in cost and time and capable to be performed at room temperature [35].
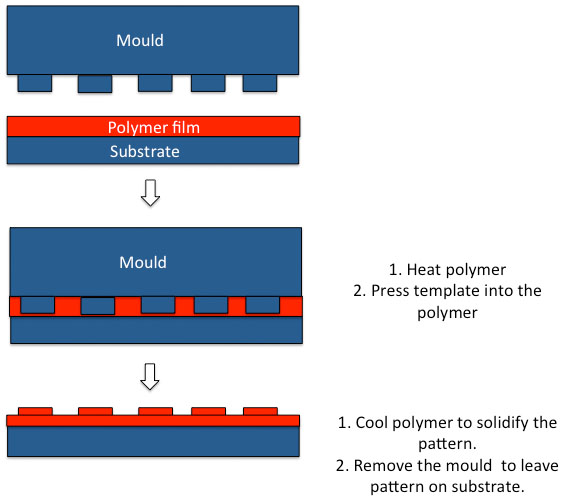
Nanoimprinting has been used in tissue engineering to pattern 3D scaffolds and to investigate cell responses. For example, Wu et al. utilised thermal nanoimprinting to develop spatially controlled nano topography in the form of nanopillar, nano-hole and nano-grill on polycaprolactone (PCL) [38]. Certain nano-topographical patterns triggered the differentiation of the human mesenchhymal stem cells and changes in their cytoskeletal arrangement and cell aggregation [38]. The authors found that non-patterned surface, nano-pillar and nano-hole topography enhanced MSC chondrogenesis and allowed the formation of hyaline cartilage [38]. Nanogrill surfaces caused the MSCs to produce fibro/superficial zone cartilage. This study highlights the importance of topographical design in scaffold for cartilage tissue engineering [38].
Modification of materials surfaces using RGD peptide, a transmembrane ligand has shown to regulate cell adhesion and widely used in regenerative medicine to optimise tissue regeneration [39]. The improvement of RGD effects and chondrogenesis has been extensively reported [39]. Using nanolithography, hexagonal RGD patterns on PEG hydrogels was investigated on the chondrogenic differentiation of human bone marrow derived stem cells (hBMSCs) [39]. The spacing of RGD patterns was investigated, using two nanospacing (63 and 161 nm) on the differentiation of the BMSCs. Large nanoscale patterning led to a decrease in the spreading and a higher chondrogenic differentiation as shown by expression of collagen II and chondrocyte specific genes (SOX-9, aggrecan and collagen II) [39].
DIP PEN LITHOGRAPHY
Another technique, which has been shown to be able to create nanostructured surface is dip pen lithography (DPN) [32]. This is another technique, which has become a method to create sub-100 mm scale dimensions onto substrate, which is based on atomic force microscopy (AFM) [32]. However, the high cost, limited substrate materials and need for a flat surface means that it has limited capabilities at present. To date, DPN has shown to construct arrays of proteins and used to study cell-protein interactions.
COATINGS
Many of the techniques discussed have patterned surfaces on the material directly to create a nanotopography to influence cell behaviour. An alternative method is to graft bioactive peptide or proteins onto the implant surface to create a specific nanootopography to direct cell responses. Several techniques can be utilised to control the architecture of the spacing of the signalling modulus to ensure tissue regeneration [40-43]. Surfaces can be modified with different biomolecules, peptides and DNA molecules to create nanotopographies [43]. Techniques used to functionalise the material and immobilise the molecules onto the surface can vary from chemical methods, which include chemical reactions such as hydrolysis or aminolysis, using plasma surface modification to create reactive surfaces or using UV radiation, which incorporates chemical functional groups onto the surface which can be used for tissue regeneration [43]. To allow for the attachment of growth factors onto scaffolds surfaces can utilise covalent bonding strategies or physical adsorption [43].
Few studies have utilised the immobilisation of RGD to enhance the chondrogenic differentiation of stem cells [44]. Chemical immobilisation of RGD peptide demonstrated the chondrogenic differentiation of human tooth germ stem cells. Poly(γ-benzyl-l-glutamate) (PBLG) scaffolds underwent a two-stage reaction using ‘snap’ chemistry to immobilise RGD peptide sequence . The scaffolds were found to be suitable for growth and differentiation of the stem cells [44]. Similarly, human umbilical cord derived stem cells have shown the chondrogenic differentiation on poly (3-hydroxybutyrate-co-3-hydroxyhexanoate) (PHBHHx) scaffolds coated with polyhydroxyalkanoate binding protein fused with arginyl-glycyl-aspartic acid (PhaP-RGD) [45]. Alternatively, laminin derived peptide has shown to promote the attachment and proliferation of adipose derived stem cells on PCL scaffolds by covalent bonding using carbodiimide chemistry [46].
Proteins have been thoroughly investigated to improve the biocompatibility of surfaces for cartilage regeneration. For example, grafting of collagen and basic fibroblast growth factor was also shown to promote the chondrocyte spreading and growth on poly-L-lactide (PLLA) scaffolds using water-soluble carbodiimide chemistry [47]. Grafting of nanostructures has recently been investigated to recreate the microenvironment. Carbon nanotubes are nanocylinders of carbon atoms that have good mechanical, electrical and thermal conductive properties [48]. Grafting of single wall carbon nanotubes has also been investigated for cartilage tissue engineering. Chondrocytes tolerated the single walled nanotubes (SWNTs) well, with minimal toxicity of cells in 3D culture [49]. There was an increase in the GAG content in SWNT- Polyethylene glycol (PEG) and SWNT-COOH compared to the control [49]. Surface coating also increased the collagen type II and fibronectin expression, suggesting there was a promotion of ECM expression [48].
In addition, to the above techniques used to create nanotopographies these techniques can nanopattern peptides and proteins on surfaces [49, 50]. The first technique that can impart peptides is by using self assembled monolayers (SAMs) [49]. These are long molecules, which at the end have a high affinity for a specific substrate and a tail, which has a specific functional group [49]. These SAMs can be functionalised with peptides and trigger certain cell responses [49]. Microcontact printing described earlier can also be used to pattern surfaces with peptides [50]. The stamp is dipped in ink, a peptide, which is later transferred onto the contact substrate [50]. Nanoimprinting lithography can also be used to pattern peptides onto surfaces [51]. A silicone nanotemplate is imprinted on to the substrate using a polymer as described above [51]. The residual polymer on the imprinted surface is then etched to create an inverse pattern on the underlying surface, on which a reactive layer is laid down which create a protein nanofeature [51]. Atomic force microscopy (AFM) based techniques can also be used not only to create topographies but peptide patterning [52]. Instead of the AFM tip creating a nanotopography the AFM is dripped in a protein solution, which is directly written onto the substrate surface [52]. Indirect methods include dipping the AFM tip in a solution of protein adherent SAM molecules [52]. The surface is the incubated with a protein, which adsorbs highly on area where the SAMs molecules were nanopatterned [52]. Nanoscale patterning of peptides is early development but offers several advantages in controlling cell behaviour [52]. Nanopatterning of peptides enables the tighter control of proteins delivery retaining the full bioactivity of the signalling molecules and also the presentation of a much density of peptides than with traditional techniques [52].
FUTURE STUDIES
Surface geometry determines the arrangement of cell cytoskeletal proteins, which can regulate cell adhesion and cause signals for differentiation. Therefore, techniques to impart nanoscale sized features on implant surfaces have become a tool for tissue regeneration. Techniques used to pattern surfaces for regeneration of tissues have been explored over the last decade, allowing the manufacture of nanostructures on the surfaces of implants.
To date it is clear that many of the simple chemical surface treatments to impart nanotopographies have been researched for bone tissue engineering. Further, research is required for using simple chemical modification including acid and alkali treatments to optimise chondrocyte adhesion and chondrogenic differentiation. Physical methods have been started to be investigated to optimise cartilage adhesion including plasma surface modification. However, the exact plasma treatment to impart topography to increase chondrocyte adhesion is unknown and deserves further research development. The effect of different nanocoatings on materials has been shown to promote chondrocyte adhesion, proliferation and differentiation. However, the correct molecule, growth factor, peptide or proteins warrants further investigation, as this is a simple method to optimise cartilage tissue regeneration.
Nanoimprinting is a new generation of tools that can imprint 3D scaffolds with high-resolution nanofeatures. At this point it is not clear, which topography is optimal for cartilage regeneration with minimal studies investigating the effect of different topographies on chondrocyte adhesion and differentiation. It is certain that nanoimprinting will help us further understand the geometries and size of the specific geometries, which are useful for chondrocyte adhesion and differentiation. Similarly, microcontact printing and replica moulding are lithography techniques, which have been able to create nanofeatures onto substrates, which are yet to be utilised for cartilage tissue engineering.
CONCLUSION
The fast evolving field of nanotechnology has enabled nanoscale features to be patterned onto surfaces for tissue engineering and regenerative medicine. Further studies are required to understand the nanotopography characteristics to support cartilage regeneration.
LIST OF ABBREVIATIONS
ACI | = Autologous chondrocyte implantation |
AFM | = Atomic Force Microscopy |
CaP | = Calcium phosphate |
DBM | = Demineralised bone matrix |
DPN | = Dip pen lithography |
ECM | = Extracellular Matrix Formation |
hBMDSCs | = Human Bone Marrow Derived Stem Cells |
PCL | = Polycaprolactone |
PDMS | = Polydimethylsiloxane |
PEG | = Polyethylene glycol |
PLLA | = Poly(lactic acid) |
SAM | = Self assembled monolayers |
sGAG | = Sulphated glycosaminoglycans |
SWNTs | = Single walled nanotubes |
UV | = Ultraviolet |
CONFLICT OF INTEREST
The authors confirm that this article content has no conflict of interest.
ACKNOWLEDGEMENTS
We would like to thank the funding from Medical Research Council and Action Medical Research, which provided MG with a clinical fellowship, GN 2339, to conduct this work.