All published articles of this journal are available on ScienceDirect.
In Vivo Bioluminescence Imaging – A Suitable Method to Track Mesenchymal Stromal Cells in a Skeletal Muscle Trauma§
Abstract
Cell-based therapies have emerged during the last decade in various clinical fields. Especially mesenchymal stromal cells (MSCs) have been used in pre-clinical and clinical applications in cardiovascular, neurodegenerative and musculoskeletal disorders. In order to validate survival and viability as well as possible engraftment of MSCs into the host tissue a live cell imaging technique is needed that allows non-invasive, temporal imaging of cellular kinetics as well as evaluation of cell viability after transplantation. In this study we used luciferase-based bioluminescence imaging (BLI) to investigate the survival of autologous MSCs transplanted into a severely crushed soleus muscle of the rats. Furthermore we compared local as well as intra-arterial (i.a.) administration of cells and analyzed if luciferase transduced MSCs depict the same characteristics in vitro as non-transduced MSCs. We could show that transduction of MSCs does not alter their in vitro characteristics, thus, transduced MSCs display the same differentiation, proliferation and migration capacity as non-transduced cells. Using BLI we could track MSCs transplanted into a crushed soleus muscle until day 7 irrespective of local or i.a. application. Hence, our study proves that luciferase-based BLI is a suitable method for in vivo tracking of MSCs in skeletal muscle trauma in rats.
INTRODUCTION
Cell-based therapies started as a regenerative modality from pre-clinical analyses and now entered clinical phase analyses in various application fields. Especially mesenchymal stromal cells (MSCs) are proposed for clinical application in cardiovascular, neurodegenerative and musculoskeletal disorders [1-3]. Besides the therapeutic benefits of MSC treatment, a clear mode of action yet has to be resolved. In addition, it remains unclear if the modes of action that are discussed are identical for all cell types in various clinical applications. MSCs seem to improve among others (a) symptoms in neurodegenerative diseases by secretion of anti-inflammatory cytokines [4], (b) functional regenerating of myocardial tissue by a pro-angiogenic stimulation of the hosting tissue [3], and (c) functional regeneration of skeletal muscle tissue by means of a fiber type shift which results in higher contraction forces [5]. Nevertheless, it remains unclear at what moment after application and by which specific modes of action (a cell-cell interaction or mainly a paracrine stimulus or an immune-modulatory effect) the MSCs by themselves modulate the situation at the site of injury and thus enable regenerative cascades. Evaluation of cell based strategies rely primarily on analysis of the tissues at specific time points after explantation e.g. by means of histology, gene expression analysis and characterization of the immunological statuses or functional testing of tissues [4, 6, 7]. So far, time course analyses of cellular recruitment, the validation of cellular survival or even the analysis of MSC engraftment into host tissues cannot be observed satisfactorily [8, 9]. To follow the time course or even characterize cells in the in vivo setting, modalities for live cell imaging and tracking are mandatory to enable non-invasive, temporal imaging of cellular kinetics as well as evaluation of cell viability after transplantation.
Bioluminescence imaging (BLI) may be a technique that could enable such in vivo imaging: the technique becomes more and more popular in pre-clinical analysis to address the above-mentioned aspects. Apparently it allows non-invasive imaging over a longer period in vivo and in addition gives information on the viability of cells [10, 11].
Approaches on stem cell tracking in vivo have been reported, e.g. for the treatment of myocardial infarction (see review of de Almeida, 2011) [11], in ischemic brain or spinal cord injury [12, 13] and in muscle injury [9]. In a rat model of ischemic brain injury human neural stem cells were labeled with iron oxide nanoparticles and tracked via MRI. A major drawback is that a considerable loss of signal due to dilution of iron oxide nanoparticles by means of e.g. cell division or possible artifacts due to uptake of particles by phagocytes challenges the precision of this approach [12]. To investigate the in vivo distribution of MSCs transplanted into a crushed skeletal muscle, a study by Winkler et al. [9] using MSCs labeled with very small iron oxide nanoparticles (VSOPs) was initiated. In vivo visualization was done using MRI after cell transplantation until day 42. Clear MRI - signals of the VSOPs could be obtained over the observation period. However, histological staining also showed that VSOPs were taken up by macrophages that reside in the crushed soleus muscle. Even though MRI has a very high resolution that allows spatial analysis of transplanted cells as well as anatomic features it does not give any information about the viability of the MSCs. Thus it can only be estimated how long MSCs survive in the highly inflammatory environment of a crushed skeletal muscle. In a spinal cord injury model in mouse, Ozedmir et al. [12]. tracked bone marrow mononuclear cells and MSCs labeled with luciferase. The authors report a drastic reduction of the bioluminescence signal within the first week for various cell types. The most promising approach was reported by Evereart et al. [14]. They reported an MSC visualization that aimed at monitoring the homing of these cells to ischemic tissue in a hind limb mouse model using BLI after different injection strategies. The bioluminescence signal was detectable until day 8 after transplantation though ischemia-directed homing of MSCs after systemic application could not be observed.
In contrast to the approaches reported above, an in vivo imaging using luciferase has so far not been realized to document cellular behavior or survival in a severely crushed soleus muscle. In vivo imaging using luciferase allows real-time monitoring of survival and migration/homing of MSCs [11]. Furthermore, a conclusion about the viability of the transplanted MSCs can be drawn, since bioluminescence can only be detected if these cells are able to produce ATP, hence are viable [10].
Even though some studies were conducted tracking stem cells using in vivo BLI in various animal models, no BLI imaging has been done of MSCs transplanted into an injury model such as a crushed skeletal muscle.
The aim of our project was to track MSCs in vivo after local as well as intra-arterial (i.a.) transplantation into an injured skeletal muscle, and to evaluate viability and migration behavior using these different administration routes of cells. Furthermore we wanted to analyze if luciferase transduction altered the in vitro characteristics of the cells in terms of their differentiation, proliferation and migration potential.
MATERIALS AND METHODS
In Vitro Differentiation Assays of MSCs
To validate that luciferase does not alter cellular differentiation of rat bone marrow MSCs, cells obtained from tibial biopsies (see below) were transduced via a lentiviral system to insert dscopGFP (Lentivector Expressing Systems; System Biosciences, Mountain View, California, USA). Transduction was performed by adding virus, produced in a T293 HEK producer cell, to adherent MSCs. GFP was used as transduction control. Osteogenic and adipogenic differentiation potential as well as proliferation was assessed as follows. Luciferase, GFP and non-transduced MSCs were divided into three groups and cultured in osteogenic or adipogenic medium and expansion medium. Osteogenic medium contained 200μM ascorbic acid, 7mM β-glycerophosphate and 0.01μM dexamethasone. Adipogenic medium contained 1μM dexamethasone, 2μM insulin, 200μM indomethacine and 500μM isobutylmethyl-xanthine. Expansion medium, i.e. Dulbecco's Modified Eagle Medium (DMEM, Gibco, Paisley, Great Britain) supplemented with 10% fetal calf serum (Biochrome, Berlin, Germany), 1% Glutamine (Gibco, Paisley, Great Britain) and 1% Penicillin-Streptomycin (Sigma, Taufkirchen, Germany), was used as a negative control for differentiation. Differentiation assays were performed over a period of 21 days at 37°C and 5% CO2. 2.4x104 cells per well were stimulated with osteogenic or adipogenic media and stained with Alizarin Red to quantify osteogenic and Oil Red for quantification of adipogenic differentiation. Obtained values for Alizarin Red and Oil Red staining were normalized to cell number determined by Alamar Blue. Proliferation was assessed using Alamar Blue (Promega, Madison, WI, USA) according to manufactures protocol.
In Vitro Migration Assay
In order to characterize the alteration of MSC migration due to luciferase, the in vitro migration potential of MSCs was characterized by time-lapse microscopy with and without luciferase as well as GFP using Ibidi cell culture inserts (Ibidi, Munich, Germany). 1.1x104 MSCs were seeded into each compartment of the device and grown to confluence at 37°C and 5% CO2 in Dulbecco's Modified Eagle Medium (DMEM, Gibco, Paisley, Great Britain) supplemented with 10% fetal calf serum (Biochrome, Berlin, Germany), 1% Glutamine (Gibco, Paisley, Great Britain) and 1% Penicillin-Streptomycin (Sigma, Taufkirchen, Germany). The cell culture insert was removed, cells were washed with PBS and fresh media were added. Live cell imaging was performed over a period of 48h using the DMI6000B Leica Life cell imaging system and LAS AF Lite software (both Leica Mikrosysteme, Wetzlar, Germany). Images were analyzed using Tscratch software (http://www.cse-lab.ethz.ch.) [15].
Animals and Experimental Setup
10 female Sprague Dawley rats (140 – 160 g, Charles River, Sulzbach, Germany) were used in this study. Animals were housed at a constant temperature with free access to food and water. All animal experiments were approved by the German local authorities and were conducted in accordance with the Guide for the Care and Use of Laboratory Animals by the National Academy of Science, Washington D.C. (2001). Bone marrow aspirates were taken from both tibiae of each animal on day 0. Three weeks later the left soleus muscle was bluntly crushed to mimic muscle injury. Seven days after injury MSCs were either transplanted locally into the soleus muscle (n=5) or into the femoral artery (i.a., n=5). In vivo data of the transplanted cells were obtained by examination of the animals in an IVIS® Lumina Imaging System (Xenogen Corporation, Alameda, CA, USA). Bioluminescence imaging (BLI) was carried out on day 1, 4 and 7 days post transplantation for both groups.
Bone Marrow Biopsy and Isolation of MSCs
Bone marrow biopsies were performed as described before [6]. In brief, rats were anesthetized with 2,5 % isoflurane. A longitudinal incision was made medially of the tibial tubercle. The cortical bone was opened using a 1 mm manually driven drill. Bone marrow was aspirated twice with an 18-gauge canula and stored in 10mL of Dulbecco's Modified Eagle Medium (DMEM, Gibco, Paisley, Great Britain) supplemented with 10% fetal calf serum (Biochrome, Berlin, Germany), 1% Glutamine (Gibco, Paisley, Great Britain) and 1% Penicillin-Streptomycin (Sigma, Taufkirchen, Germany). Aspirates were seeded and cultured at 37°C and 5% CO2 and expanded for three weeks. Cells no later than passage four were used for the experiments.
Muscle Trauma and Transplantation of MSCs and BLI
Animals received a standardized muscle trauma as described before [6]. In brief, a posterolateral longitudinal incision of the skin and underlying fascia was made at the left lower limb. The soleus muscle was mobilized from the lateral gastrocnemius head to the Achilles tendon. The muscle was crushed three times distally and two times proximally to the neurovascular bundle, using a curved artery forceps (Aesculap, Tuttlingen, Germany). The tips were protected by polyethylene tubes. After surgery the skin was sutured. One week after trauma transplantation of luciferase transduced MSCs was performed. Cells were harvested, washed and 2.5x106 cells were transplanted either locally into the soleus muscle or into the femoral artery. For in vivo imaging fresh stock solution of 15mg/ml D-Luciferin in DPBS was prepared and sterilized through a 0.2µm filter and 800µl D-Luciferin were injected intra-peritoneal according to manufacturer’s protocol. Imaging was done 10-15 min after injection of D-Luciferin at maximum signal intensity. Animals were imaged under anesthesia with an IVIS® Lumina Imaging System (Xenogen Corporation, Alameda, CA, USA) using 5 min exposure time.
Statistical Analysis
The mean and standard error were determined for each parameter. A statistical analysis was performed using one way Anova. The level of significance was set to 0.05.
RESULTS
In Vitro Proliferation and Differentiation Potential of MSCs
In order to test whether MSCs transduced with luciferase bare the same proliferation and differentiation potential as non-transduced MSCs, we performed adipogenic and osteogenic differentiation assays and analyzed proliferation of these cells.
Fig. (1) shows the quantification of osteogenic and adipogenic differentiation of GFP and luciferase transduced MSCs as well as non-transduced MSCs as control. Osteogenic differentiation could be observed in untreated cells and MSCs cultured in osteogenic medium (Fig. 1a, mean value extinction [Alizarin Red/Alamar Blue] control MSC untreated: 0.390 vs control MSC osteogen treated: 0.598. p= 0.002; mean value extinction [Alizarin Red/Alamar Blue] GFP MSC untreated: 0.344 vs GFP MSC osteogen treated: 0.565. p= 0.001 and mean value extinction [Alizarin Red/Alamar Blue] luciferase MSC untreated: 0.361 vs luciferase MSC osteogen treated: 0.507. p= 0.000). No significant difference in osteogenic differentiation could be observed between control and transduced MSCs (mean value extinction [Alizarin Red/Alamar Blue] control MSC: 0.598 vs GFP MSC: 0.565 vs luciferase MSC: 0.507).
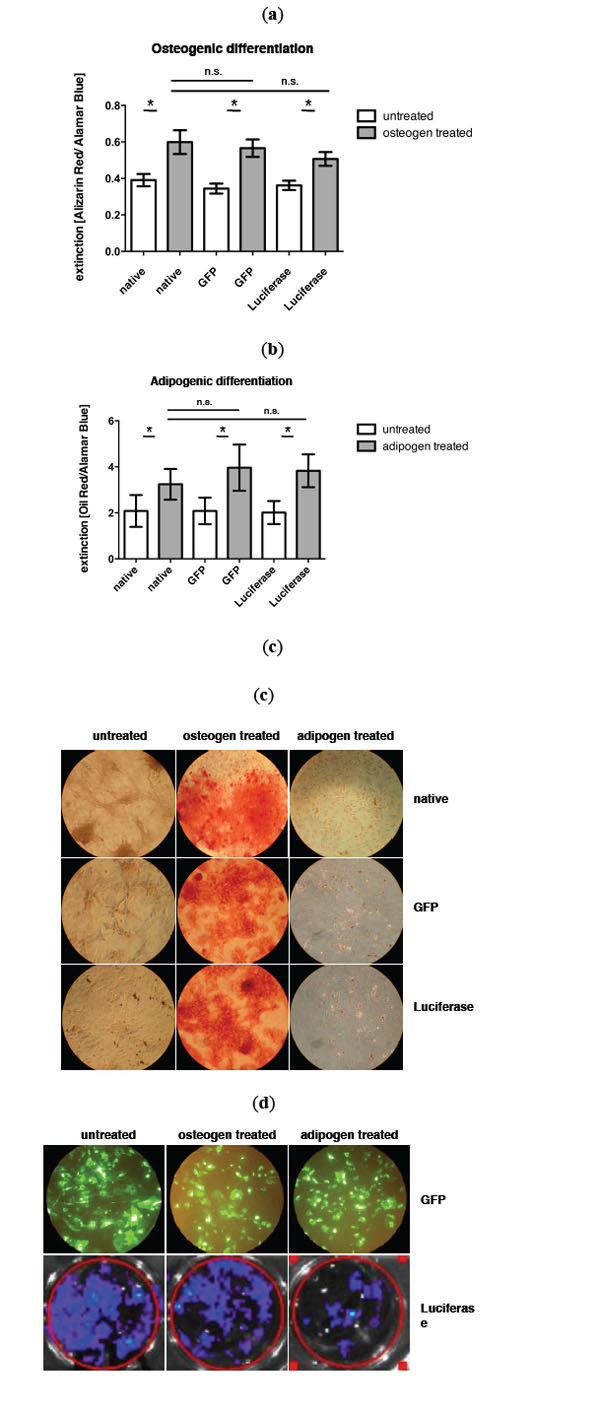
a) Quantification of osteogenic differentiation via alizarin red staining and b) adipogenic differentiation via oil red staining of nontransduced control MSCs, GFP transduced MSCs and luciferase transduced MSCs. c) Representative pictures of osteogenic and adipogenic differentiation after respective staining. d) Expression of GFP and luciferase in MSCs during differenatiation assay (day 14), upper row: BLI of luciferase expressing MSCs.
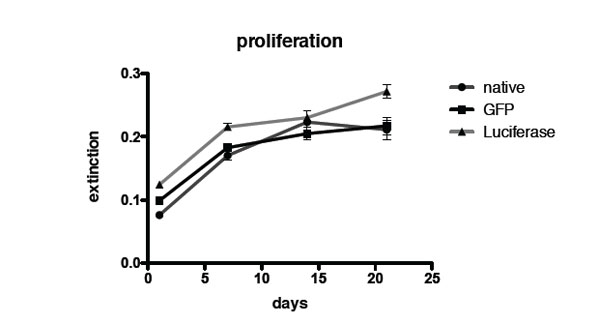
Quantification of proliferation via Alamar Blue assay of non-transduced control MSCs, GFP transduced MSCs and luciferase transduced MSCs.
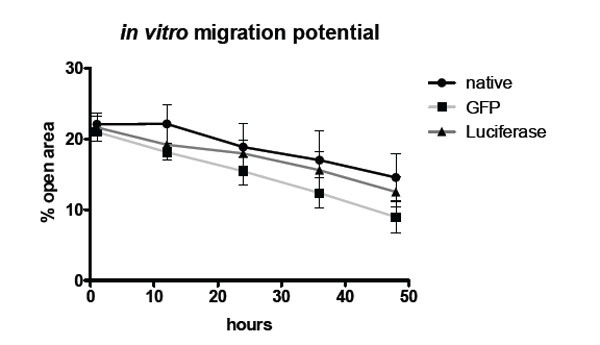
In vitro migration potential of non-transduced control MSCs, GFP transduced MSCs and luciferase MSCs.
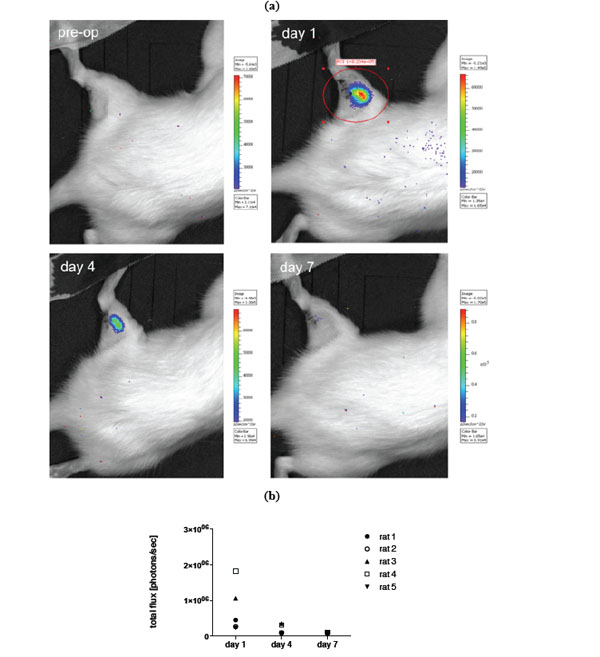
a) Example of in vivo BLI (IVIS Lumina imaging system) of luciferase transduced MSCs prior to transplantation and on day 1,4 and 7 after local transplantation into a crushed injury of the soleus muscle. b) Quantification of BLI signal.
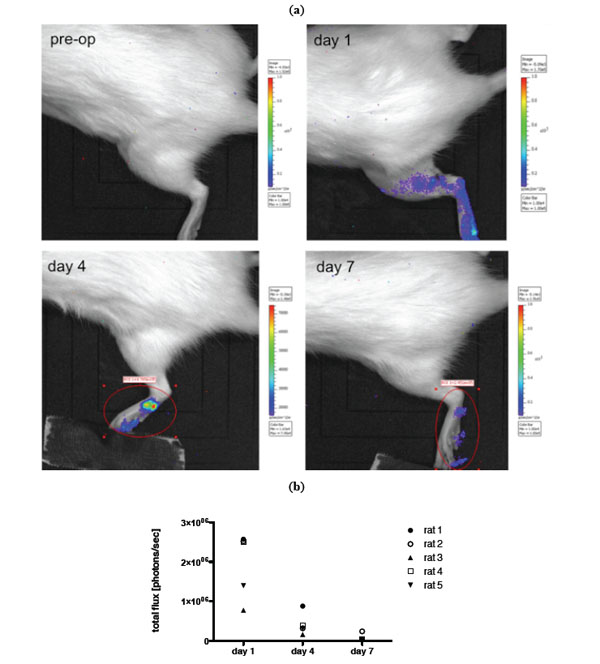
a) Example of in vivo BLI (IVIS Lumina imaging system) of luciferase transduced MSCs prior to transplantation and on day 1,4 and 7 after i.a. transplantation into a crushed injury of the soleus muscle. b) Quantification of BLI signal
Also in adipogenic differentiation a significant difference between untreated and adipogen treated MSCs could be observed (Fig. 1b, mean value extinction [Oil Red/Alamar Blue] control MSC untreated: 1.962 vs control MSC adipogen treated: 3.253. p= 0.009; mean value extinction [Oil Red/Alamar Blue] GFP MSC untreated: 1.969 vs GFP MSC adipogen treated: 3.939. p= 0.015 and mean value extinction [Oil Red/Alamar Blue] luciferase MSC untreated: 2.012 vs luciferase MSC adipogen treated: 3.829. p= 0.002). No significant difference in the adipogenic differentiation potential between control and transduced MSCs could be measured (mean value extinction [Oil Red/Alamar Blue] control MSC: 3.253 vs GFP MSC: 3.939 vs luciferase MSC: 3.829). In Fig. (1c) the expression of GFP and luciferase during differentiation is exemplarily shown on day 14.
Fig. (2) shows quantification of proliferation using Alamar Blue assay. No difference in the proliferation rate between transduced and non-transduced MSCs and GFP labeled MSCs could be seen.
In Vitro Migration Potential of MSCs
Comparison of non-transduced MSCs and transduced MSCs reveals no significant differences regarding the migration potential. Fig. (3) shows the quantification of the migration assay using Ibidi cell culture inserts. Displayed is the percentage of open area in the well that is covered by cells over time.
In Vivo Fate of MSCs
For in vivo imaging BLI was done on day 1, 4 and 7 post transplantation of MSCs. Images of an animal after local and i.a. transplantation (TX) are shown in Figs. (4, 5). Results of imaging data are summarized in Fig. (4b) as well as (5b). Over the period of seven days a clear decline in the signal could be observed in all animals irrespective of the transplantation route (mean value total flux [protons/sec] day 1: 9.50x105vs day 7: 9.62x104 for local TX and mean value total flux [protons/sec] day 1: 1.74x106vs day 7: 1.04x105 for i.a. TX). No bioluminescence signal could be detected beyond day 7. Further, no cellular accumulation in the lungs, spleen and other areas of the animals was detected. Notably the bioluminescence signal in the local transplantation group is very heterogeneous and intensities on day 1 are significantly lower compared to the i.a. transplantation group (Fig. 4b, 5b).
Interestingly a bioluminescence signal could still be detected in vitro beyond two weeks (Fig. 1b). Also during differentiation the bioluminescence signal as well as the GFP signal was not lost.
DISCUSSION
This study aims at investigating how long MSCs are viable and traceable after transplanting them into a traumatized skeletal muscle in vivo. We used luciferase transduced cells and compared local and i.a. TX of these cells. A severe crush trauma of the soleus muscle in rat was used, that has previously been established as a standard animal model to monitor muscle regeneration with and without stem cell transplantation. In previous studies we could show that both ways of cell administration lead to an increase of muscle force after 3 weeks of transplantation [6, 16]. In the presented study we used bioluminescence imaging to track MSCs in vivo and to gain information about their viability after transplantation. Transplanted MSCs, independently of their administration route, could only be tracked until day 7 after transplantation. Furthermore, the bioluminescence signal declined rapidly after day 1 in both groups. However, bioluminescence signals in the local transplantation group were significantly lower compared to the i.a. transplantation group (Figs. 4, 5). This might be due to the different surroundings the MSCs had been transplanted into. Direct transplantation into an inflamed tissue, i.e. the traumatized muscle, might result in increased cell death, since there was a phase of progressive destruction of the muscle tissue. The blood stream might be a more favorable environment for MSC injection, since cell survival was higher even though half the cells had been cleared from the blood stream within 3 days (Fig. 5). Thus, a decline in the signal is presumably due to decrease in viability or number of MSCs.
Regarding the survival of stem cells in vivo, similar observations have been made in other studies. In a recent study of Nakabayashi et al. [17]MSCs were locally transplanted into an injured tibialis anterior muscle and a rapid decline in bioluminescence signal was observed after 3 days as well as very little signal after 7 days. Min et al. [18] found a diminished bioluminescence signal within 8 days after transplantation of cord blood derived MSCs into the intact myocardium in rats. Also, Zhuo et al. [19] found a decline of the bioluminescence signal of MSCs until day 7 in rats investigating an ischemia/reperfusion-induced acute renal failure model. However, the survival of stem cells seems to be highly dependent on the environment they are transplanted into and also on the cell type. Daadi et al. [12] could detect bioluminescence signals from embryonic stem cells in an ischemic rat brain until 8 weeks after transplantation. Also the animal model itself has a major impact on the outcome of cell therapy. Geuze et al. [20] showed very impressively that after ectopic transplantation of MSCs in mice a bioluminescence signal was observed over the whole period of the study of 42 days in comparison with rats in which the bioluminescence signal declined rapidly after day 14 even though the transplantation setup remained the same. The reason for this contradictory outcome remains unclear. A decreased signal due to a detection limit can be ruled out, since skin and muscle have the highest transmission and penetration depth of the bioluminescence signal is up to 2 cm [21].
Our results indicate that MSCs are more likely to contribute to an improved functional outcome only within the first week. Results from this present study as well as increased muscle force after MSC – TX, as shown before, support the hypothesis that MSCs contribute to an improved healing of tissue rather through a secretory effect, since differentiation does not take place within one week as also shown in vitro [22]. Furthermore, we could see in the present study that after i.a. TX MSCs rather get trapped in the capillaries of the paw than actively migrate to the site of inflammation; in this case the traumatized soleus muscle. These observations also favor the hypotheses of a secretory effect of MSCs that contribute to a better physiological outcome.
Nevertheless, tracking MSCs in vivo by using luciferase is a valuable tool for investigating cell based therapies. The bioluminescence signal allows assessing the survival and viability of MSCs since the conversion of the substrate D-luciferin is dependent on the intrinsic ATP of the cell. If ATP is not available D-luciferin will not be processed. Thus, no photons will be released [10]. However, using this method one has to consider the species as well as the model that is used, since bioluminescence signals seem to decline more rapidly in rat models than in mice models. This has to be taken into account especially when comparing different animal models or transferring cell transplantation strategies from one animal model into the other. Furthermore, the application strategy of cells has to be chosen carefully. Cells are cleared differently when injected into the blood stream or transplanted locally, which could possibly influence the outcome of the therapy.
CONCLUSION
To our knowledge the presented data could show for the first time that transplanted MSCs can be tracked via bioluminescence imaging signal until day 7 irrespective of local or i.a. TX following a skeletal muscle trauma. The in vitro results prove that the transduction of MSCs with luciferase does not alter their characteristics regarding differentiation, proliferation and migration capacity. Thus, these cells should bare the same properties in vivo as non-transduced MSCs, making bioluminescence imaging a suitable method for in vivo tracking of MSCs in skeletal muscle trauma in rats.
ABBREVIATIONS
BLI | = Bioluminescence |
GFP | = Green fluorescent protein |
i.a. | = Intra-arterial |
MSC | = Mesenchymal stromal cells |
TX | = Transplantation |
CONFLICT OF INTEREST
The authors confirm that this article content has no conflict of interest.
ACKNOWLEDGEMENTS
We thank Yasmin Müller for providing excellent technical assistance and Dr. Petra Schulz as well as Dr. Carsten Grötzinger for their kind help with the bioluminescence imaging.