All published articles of this journal are available on ScienceDirect.
Effect of Platelet-Rich Plasma on Chondrogenic Differentiation in Three-Dimensional Culture
Abstract
Platelet-rich plasma (PRP) may have the potential to enhance articular cartilage regeneration through release of growth factors including transforming growth factor isoforms. The purpose of this study was to investigate the potential for PRP to stimulate chondrogenic differentiation in three-dimensional PRP hydrogel constructs. Allogenic PRP was prepared using a double centrifugation protocol which resulted in a platelet concentration approximately 250% above baseline. Canine marrow stromal cells were encapsulated at 6.8×106 cells/ml in either 2% sodium alginate or in a 3:1 mixture of freshly prepared PRP and 2% alginate. PRP and alginate beads were cultured in chemically defined chondrogenic medium with and without 10 ng/ml TGF-β3. PRP cultures were additionally supplemented with frozen-thawed PRP. In the absence of TGF-β3, PRP had a mild stimulatory effect on cell proliferation. PRP did not stimulate cell proliferation in the presence of TGF-β3. Cells exposed to TGF-β3 accumulated significantly more GAG/DNA than those which were not, but there was not a statistically significant difference between alginate and PRP. Total collagen content was greater in PRP than in alginate, regardless of TGF-β3. Chondrogenesis in PRP was qualitatively and spatially different than that which occurred in conventional alginate beads and was characterized by isolated centers of intense chondrogenesis. Overall the results demonstrate that PRP alone weakly promotes chondroinduction of marrow stromal cells, and the effect is greatly augmented by TGF-β3.
INTRODUCTION
Enriching the platelet concentration in blood plasma, typically through centrifugation, yields platelet-rich plasma (PRP). Each platelet carries approximately 50–80 α–granules, the contents of which are released during platelet activation [1]. These contents include hundreds of soluble proteins, including platelet derived growth factor (PDGF), transforming growth factor-beta (TGF-β), and insulin-like growth factor (IGF) [2]. Such growth factors are known to stimulate proliferation, differentiation, and migration of mesenchymal stem cells. Thus PRP could be a source of concentrated autologous growth factors with the potential to stimulate musculoskeletal tissue regeneration.
PRP has been investigated as a means to accelerate healing and enhance regeneration of bone [3, 4], tendon [5, 6], ligament [7, 8], skeletal muscle [9], and cartilage [10-13]. With respect to cartilage, the results have been mixed. Wu et al. demonstrated that subcutaneous injection of auricular chondrocytes embedded in a PRP gel produced a tissue similar to that of native cartilage in a rabbit model [13], and Milano et al. found that a PRP fibrin glue gel improved the stiffness and histological grade of repair tissue in microfracture-treated chondral lesions in the sheep stifle joint [10]. On the other hand, Kon et al. found that soaking a hydroxyapatite-collagen scaffold in autologous PRP interfered with its ability to support osteochondral regeneration [12].
Our long-term goal is to clarify the potential for PRP to improve approaches to cartilage tissue engineering based on chondroinduction of marrow-derived mesenchymal stem cells (MSCs). Several studies have shown that PRP stimulates proliferation of MSCs in monolayer [14-16], and the PRP-induced expansion does not diminish the MSCs’ multilineage differentiation potential [17]. Some studies demonstrated that PRP can accelerate osteogenic differentiation in vitro, as evidenced by PRP-induced increases in alkaline phosphatase and mineralization [18-20], whereas others have observed no osteoinductive effect of PRP [21, 22]. There are far fewer published reports concerning the effect of PRP on chondrogenic differentiation, but the literature to date is somewhat encouraging. Mishra and colleagues demonstrated that non-activated PRP substantially upregulated gene expression of the chondrogenic markers Sox-9 and aggrecan in monolayer cultures of human MSCs [23]. Krüger et al. observed that exposure of human cortico-spongious progenitor cell pellets to frozen/thawed PRP increased proteoglycan and type II collagen staining and induced gene expression of aggrecan, collagen types II and IX, and link protein [24]. However, a study by Drengk and coworkers demonstrated an inhibitory effect of PRP on chondroinduction of ovine bone marrow MSCs in pellet culture [25]. The addition of PRP to culture medium containing 10% fetal bovine serum negatively influenced immunhistochemical staining for type II collagen.
The purpose of the current study was to investigate the potential for PRP to stimulate chondroinduction of MSCs in three-dimensional hydrogel culture. We hypothesized that cells embedded in PRP/alginate hydrogels and exposed to frozen-thawed PRP in the culture medium would exhibit superior chondroinduction to cells in alginate beads without PRP supplementation. A standard method of stimulating chondrogenic differentiation in vitro is by culturing MSCs in medium which contains TGF-β3 inducer. The effects of PRP were therefore tested in the presence and absence of TGF-β3 to facilitate comparison between the two and to explore the possibility that PRP could enhance TGF-β3-mediated differentiation.
METHODS
All procedures involving live animals were conducted according to protocols approved by the Mississippi State Institutional Animal Care and Use Committee. Marrow cores were harvested from the proximal femurs of 3 mixed-breed dogs using a Jamshidi needle. Pooled cores were centrifuged to isolate the marrow, which was disrupted by pipetting and seeded onto tissue culture polystyrene in Dulbecco’s Modified Eagle Medium (DMEM) containing 10% fetal bovine serum (FBS). Twenty-four hours later non-adherent cells were removed by complete medium change. Adherent cells, hereafter referred to as mesenchymal stem cells (MSCs), were expanded to the third passage.
Blood collected from 2 mixed-breed dogs was pooled together. It was collected via jugular venipuncture with a 20-gauge needle directly into 8.5 ml vacutainer tubes containing acid citrate dextrose (ACD-A) anticoagulant. Blood was centrifuged at 1200 × gravity (or 1200 g) for 3 minutes to separate red blood cells from plasma. The upper plasma phase, including the interface, was centrifuged at 1000 g for 5 minutes to pellet the platelets. Two-thirds of the platelet-poor plasma supernatant was discarded, and the platelets were resuspended in the remaining third to create platelet-rich plasma.
Half the cells were used to establish alginate bead cultures. Sodium alginate was dissolved in DMEM at 2% wt/vol and sterilized by autoclaving. MSCs were suspended in alginate at a density of 6.8×106 cells/ml, and this suspension was added dropwise to 100 mM CaCl2 in 0.9% NaCl. Following 5 minutes for polymerization, the beads were rinsed twice with phosphate-buffered saline and incubated with defined chondrogenic medium (DCM). Four wells of a 24-well plate each contained 6-8 beads and 2 ml of medium (approximately 30 beads in total). The composition of DCM was as follows: high-glucose Dulbecco’s modified Eagle’s medium (DMEM) supplemented with 1% ITS+Premix (BD Biosciences), 0.1 mM dexamethasone, 50 µg/ml ascorbate-2 phosphate, 1mM sodium pyruvate, 40 µg/ml L-proline, and 1% antibiotic-antimycotic solution (Sigma-Aldrich, St. Louis, MO). Two of the wells containing half the beads (n≈15) were additionally supplemented with 10 ng/ml transforming growth factor-beta 3 or TGF-β3 (Peprotech, Rocky Hill, NJ).
Half the cells were embedded in PRP beads. PRP was mixed with 2% alginate at a ratio of 3 parts PRP to 1 part alginate. MSCs were suspended in the mixture at a density of 6.8×106 cells/ml, and the mixture was added dropwise to a solution of 6% CaCl2 and 0.9% NaCl. Approximately 30 beads were evenly distributed to 4 wells of a 24-well plate containing 2 ml of culture medium. PRP beads were treated in the same manner as alginate beads, with half in DCM without growth factor and half in DCM containing 10 ng/ml TGF-β3 (n≈15 per group). PRP cultures were additionally supplemented with frozen-thawed PRP to 5% of the total medium volume. Media were changed every third day, including frozen-thawed PRP. Supplementation with frozen-thawed PRP was discontinued after 2 weeks.
Cell viability and chondrogenesis were assessed at 4 weeks. Cell viability was evaluated by fluorescence-based live/dead staining. A randomly selected bead from each group was stained using the PromokineLive/Dead Cell Staining Kit II (PromoCell GmbH, Heidelberg, Germany) according to the manufacturer’s instructions. Images of stained cultures were captured on an Axiovert 200M Confocal Laser Scanning Microscope (Zeiss, Göttingen, Germany). Another 2 beads per group were fixed in 10% neutral buffered formalin and embedded in paraffin for sectioning. Sections were stained with 1% Toluidine blue or immunostained using SuperPicture™ 3rd Gen IHC Kit (Invitrogen Corporation, Camarillo, CA) to detect collagen types I and II. Sections to be immunostained were deparaffinized in xylene, rehydrated in graded ethanol, and incubated with 2 mg/ml hyaluronidase in PBS for 60 minutes at 37°C. They were then incubated with 1 mg/ml pronase for 15 minutes at 37°C for antigen retrieval. A mouse monoclonal antibody to collagen type I (ab6308, Abcam, Cambridge, MA) or a mouse monoclonal antibody to collagen type II (II-II6B3, Developmental Studies Hybridoma Bank, University of Iowa) was applied for 24 hours at 4°C. Both antibodies were diluted in PBS with 1% bovine serum albumin. The type I antibody was diluted 1:100 and the type II antibody (supernatant) was diluted 1:10. Following incubation in the primary antibody slides were stained according to the instructions of the kit.
Quantitative biochemistry was performed on 10 beads per treatment group. They were digested for 24 hours in papain at 60°C according to the method of Hoemann [26]. The digestate was assayed for DNA and glycosaminoglycan (GAG) by the Hoechst and DMB methods, respectively [26]. For the Hoechst procedure, fluorescence intensity was measured using a GloMax®-Multi Jr Single Tube Multimode Reader (Promega Corporation, Madison, WI), and DNA content was determined from a standard curve created from calf thymus DNA. For the DMB procedure, optical absorbance at 530 nm and 590 nm was measured using a Micro Quant microplate spectrophotometer (BioTek Instruments, Winooski, VT), and GAG content was determined from a standard curve created with chondroitin-4-sulfate. The remainder of each sample was then freeze dried and collagen content determined using the chloramine-T assay [27]. Type I collagen from bovine skin was used as a standard. GAG and collagen contents were normalized to DNA.
Quantitative data were statistically analyzed using a univariate ANOVA model in IBM SPSS Statistics 19, and p-values less than 0.05 were taken to indicate a significant difference.
RESULTS
Platelet counts showed that PRP contained 2.5 times the concentration of platelets in whole blood. Alginate beads maintained their original shape and size while becoming decreasingly translucent. PRP beads contracted and occasionally fused together. One sample in each experimental group was examined for cell viability by live/dead fluorescence assay. All samples contained a substantial proportion of dead cells. Live cells in PRP were found in clusters, and there was not a discernible difference between the bead which had been exposed to TGF-β3 and the bead which had not. In alginate beads exposed to TGF-β3 live dead cells were evenly dispersed among live cells, and the proportions were approximately equal. In the alginate bead not exposed to TGF-β3, a single live cell was observed (Fig. 1). The individual beads randomly selected for viability staining may not have been representative of all beads subjected to the same experimental conditions.
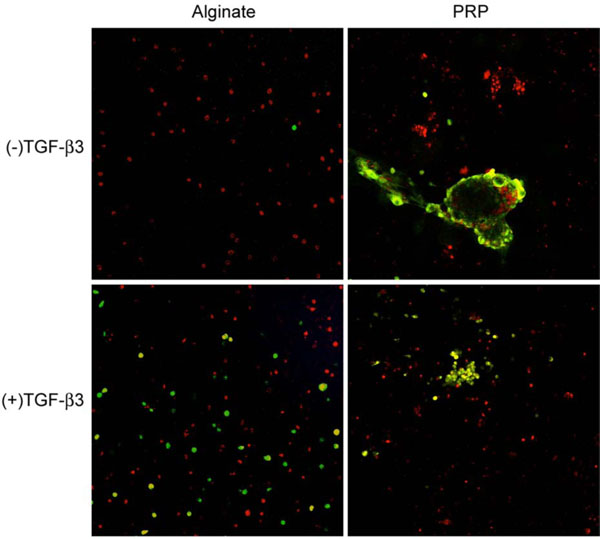
Fluorescence live-dead imaging of canine MSCs embedded in alginate and PRP and cultured 4 weeks in defined chondrogenic medium. Live cells are red and dead cells are green.
Toluidine blue staining of the cells in alginate showed them to be generally spherical. They were occasionally located within lacunae and surrounded by a pericellular metachromatic extracellular matrix (ECM). Beads exposed to TGF-β3 contained noticeably more multicellular clusters of cells surrounded by ECM (Fig. 2). In PRP without TGF-β3 some of the cells displayed the morphology of chondrocytes and had accumulated metachromatic pericellular matrix, but most did not (Fig. 3A); and there were some extensive areas which were devoid of any overt chondrogenesis (Fig. 3B). The same was generally true in the presence of TGF-β3. However, these beads also contained a few regions of the most robust chondrogenesis compared to all other groups, characterized by large cell clusters producing abundant ECM (Fig. 3C, D).
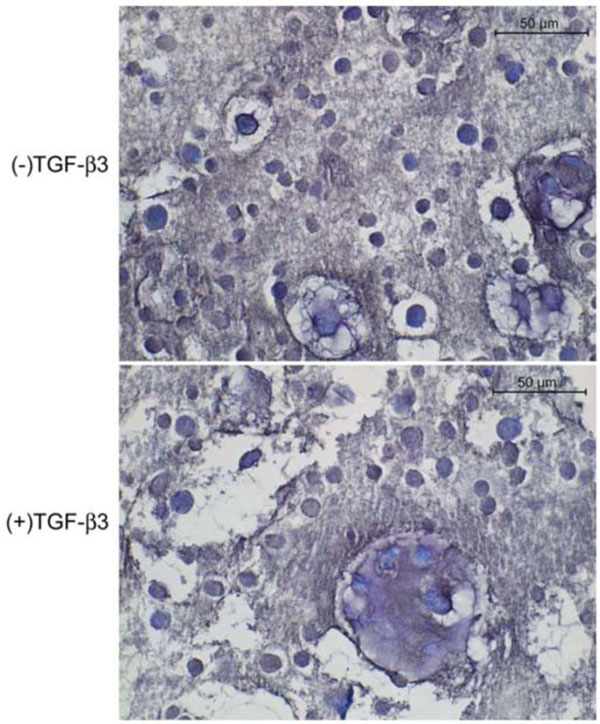
Toludine blue-stained histological sections of canine MSCs embedded in alginate and cultured 4 weeks in defined chondrogenic medium.
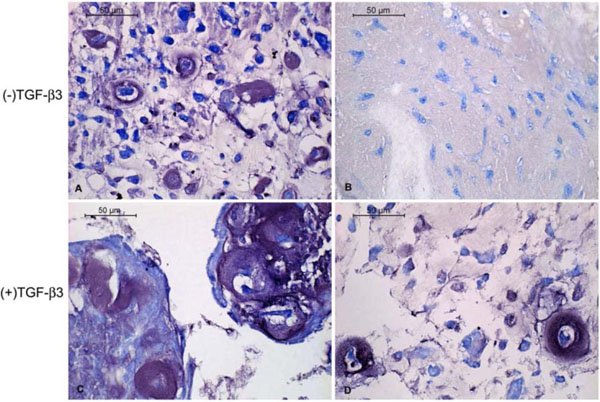
Toluidine blue-stained histological sections of canine MSCs embedded in PRP/alginate gel and cultured 4 weeks in defined chondrogenic medium. Chondrogenesis was apparent in some regions (A, C) and much less so in others (B, D).
Across all groups, dense pericellular ECM displayed intense positive staining for collagen type II (Fig. 4). Some positive staining for type I collagen could also be detected in the ECM surrounding cells in the PRP beads (Fig. 5). Sections of the alginate beads were not available for type I collagen staining.
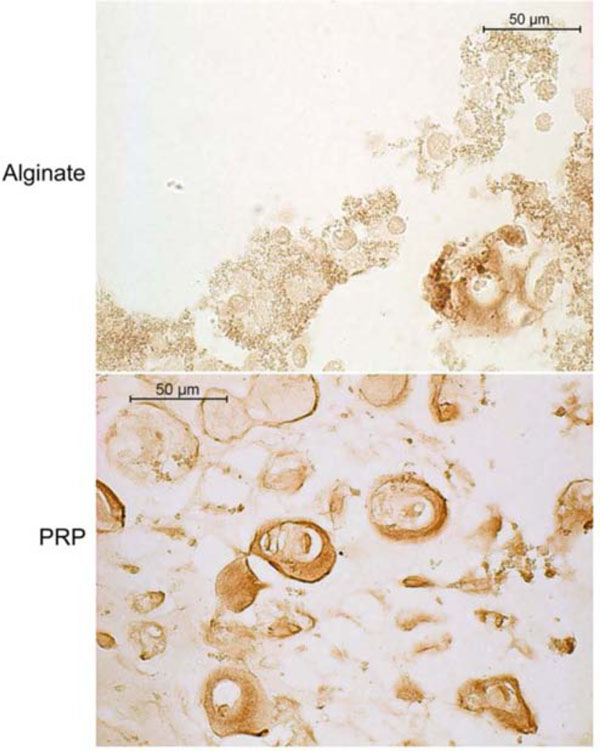
Type II collagen immunohistochemistry of canine MSCs embedded in alginate or PRP gel and cultured 4 weeks in defined chondrogenic medium containing TGF-β3. Positive staining is indicated by a reddish brown color.
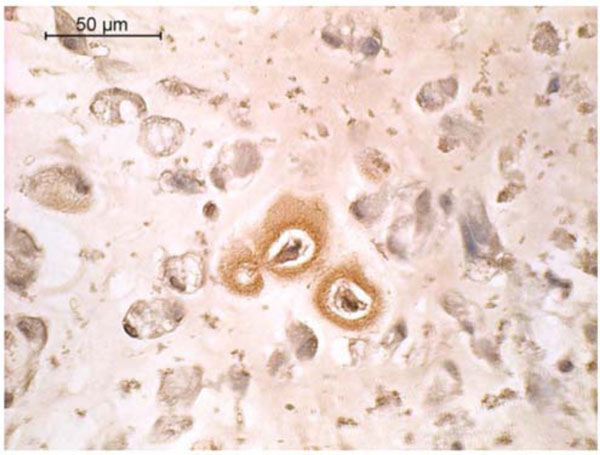
Type I collagen immunohistochemistry of canine MSCs embedded in PRP/alginate gel and cultured 4 weeks in defined chondrogenic medium without TGF-β3. Positive staining is indicated by a reddish brown color.
Biochemistry data are summarized in Table 1. In the absence of TGF-β3 PRP promoted cell proliferation, but in the presence of 10 ng/ml TGF-β3 PRP beads contained significantly less DNA (fewer cells) than alginate beads. The total amount of GAG produced by cells in alginate was greater than that produced by cells in PRP (data not shown), and TGF-β3 significantly increased GAG accumulation by cells in alginate and PRP. However, GAG content normalized to DNA content was not significantly different between PRP and alginate. One clear effect of PRP was on the amount of total collagen normalized to DNA content. It was significantly higher in PRP compared to alginate, both in the absence and presence of TGF-β3.
DNA, GAG, and collagen content of three-dimensional canine MSC cultures after four weeks of exposure to PRP and TGF-β3. Asterisks indicate a statistically significant difference between PRP and alginate (p<0.05).
Alginate | PRP | Alginate | PRP | |
---|---|---|---|---|
Without TGF-β3 | With 10 ng/ml TGF-β3 | |||
DNA (µg) | 1.08±0.14 | 1.28±0.36* | 1.20±0.16 | 0.88±0.34* |
GAG (µg/µg DNA) | 9.66±1.50 | 7.96±2.18 | 14.97±1.95 | 13.13±5.10 |
Collagen (µg/µg DNA) | 0.54±0.14 | 0.94±0.33* | 0.65±0.14 | 1.09±0.34* |
DISCUSSION
The purpose of this study was to investigate the potential for PRP to stimulate chondroinduction of MSCs in a three-dimensional culture model of the type often employed for cartilage tissue engineering. PRP is abundant in mitogenic growth factors such as TGF-β1 and PDGF and was therefore expected to increase cell proliferation in our study. In the absence of TGF-β3 the stimulatory effect of PRP on cell proliferation in hydrogel culture was similar to the previously reported effect on proliferation in monolayer culture [14-16]. However, PRP did not increase cell number when TGF-β3 was present. In fact, the addition of TGF-β3 to PRP beads seemed to suppress cell proliferation. This could have occurred because more cells were induced to switch from proliferation to differentiation toward various phenotypic lineages, including but not limited to chondrocytes.
We further assessed the ability of PRP to stimulate chondroinduction of primary canine marrow-derived mesenchymal stem cells, alone and in combination with TGF-β3. The results did not support our hypothesis. By itself, PRP was a very weak stimulus for chondroinduction and was inferior even to alginate alone. In the presence of chondroinductive TGF-β3, PRP and alginate were equivalent in terms of their overall ability to support chondrogenesis. Histology suggested there may have been a qualitative difference, though. The PRP beads contained fewer but larger foci of chondrogenesis, in which clusters of cells were surrounded by more ECM than those found in alginate. Cells in PRP may have gone through a condensation phase in the same way that mesenchymal precursors initiate cartilage development during embryogenesis, and increased cell-cell contact may have enhanced chondrogenesis. It is also possible that growth factors in the PRP were insufficient to induce differentiation but were nonetheless potent enough to stimulate ECM synthesis.
TGF-β3 was not absolutely required for chondroinduction of primary canine bone marrow MSCs, but chondroinduction was greatly enhanced by addition of TGF-β3 to MSCs in alginate and PRP. The alginate bead culture system has been demonstrated to promote efficient chondroinduction of MSCs in vitro, and was thus selected to provide a basis for comparison to PRP [28, 29]. Cells do not have receptors for alginate molecules, and they are held in a rounded shape until they synthesize a pericellular matrix with which to establish connections [30]. This spherical morphology encourages chondrogenic differentiation [31]. On the other hand, integrin-mediated cell-ECM interaction as afforded by PRP is an important aspect of TGF-β3-induced chondrogenic differentiation [32]. This might explain why the effect of TGF-β3 on chondrogenesis was more pronounced in PRP than in alginate.
It is notable that PRP beads contained significantly more collagen than alginate beads. Cartilage ECM is composed mainly of collagen and GAG, and both contribute to the tissue’s biomechanics. Tissue engineered cartilage tends to have a lower collagen:GAG ratio than native hyaline cartilage, and increasing the proportion of collagen has been shown to enhance the mechanical properties of engineered cartilage [33, 34]. Thus PRP may be beneficial for raising the collagen content of tissue engineered cartilage. The ECM surrounding cells in PRP beads contained both collagen type I and II. As tissue engineered cartilage matures in vitro, the percentage of collagen type II to total collagen steadily increases [35]. In tissue self-assembled by calf articular chondrocytes, the percentage of collagen type II to total collagen was less than 50% at 4 weeks. So the presence of collagen types I and II is consistent with that of articular cartilage neotissue grown in vitro.
The clinical efficacy of PRP for osteochondral repair remains uncertain. Promising results include the successful resurfacing of an osteochondral rabbit knee defect by synovial membrane-derived MSCs encapsulated in PRP gel and improved articular cartilage repair following intraarticular injection of muscle derived stem cells mixed with PRP in a rat model of chemically induced osteoarthritis [36, 37]. However, some results indicated that PRP has undesirable effects on chondroinduction, such as suppression of collagen type II mRNA expression by MSCs and chondrocytes in vitro [25]. Such disparate results are well documented in a recent review of in vitro and in vivo basic science studies [38]. About 70% of the studies reviewed concluded that PRP has the potential to upregulate chondrogenic gene expression as well as increase the production of proteoglycan and type II collagen, while the other studies found the effects of PRP to be negligible or disadvantageous. The total body of evidence was considered to comprise a proof of concept for the clinical use of PRP. Our data indicate that efficient chondroinduction of MSCs is not achieved with PRP only moderately enriched in platelets. The platelet concentration was only about 2.5 times that of whole blood, which may have insufficiently concentrated the growth factors platelets harbor. Increasing the platelet concentration to approximately 5-fold that of whole blood is anticipated to amplify PRP’s effects. Another limitation of the current study was that our PRP was not adjusted to neutral pH, and it did appear to decrease the pH of the culture medium based on observed changes in color of the phenol-red containing culture medium. PRP buffered to neutral pH using sodium bicarbonate has been demonstrated to enhance MSC proliferation and chondrogenic differentiation [23].
CONCLUSION
Although the current study did not demonstrate a strong chondroinductive effect of PRP on marrow-derived MSCs, the results suggest that it may stimulate collagen production and enhance the chondrogenic effect of exogenous TGF-β3.
CONFLICT OF INTEREST
The authors confirm that this article content has no conflict of interest.
ACKNOWLEDGEMENTS
The II-II6B3 monoclonal antibody developed by T.F. Linsenmayer was obtained from the Developmental Studies Hybridoma Bank developed under the auspices of the NICHD and maintained by The University of Iowa, Department of Biology, Iowa City, IA 52242.