All published articles of this journal are available on ScienceDirect.
Pathophysiology and Biomechanics of the Aging Spine
Abstract
Aging of the spine is characterized by two parallel but independent processes: the reduction of bone mineral density and the development of degenerative changes. The combination of degeneration and bone mass reduction contribute, to a different degree, to the development of a variety of lesions. This results in a number of painful and often debilitating disorders. The present review constitutes a synopsis of the pathophysiological processes that take place in the aging spine as well as of the consequences these changes have on the biomechanics of the spine. The authors hope to present a thorough yet brief overview of the process of aging of the human spine.
The human spine is a modular structure, whose role is to protect neural elements, to support the trunk in the upright position and allow motion [1]. From the axis to the sacrum, it is composed of alternating subunits, the bony vertebrae and the fibrocartilagenous intervertebral disks. The decrepitude of the spine causes changes in the structure as well as the shape of its parts, which result in encroachment and irritation of the very same neural elements it should protect, degradation of trunk supporting capability and limitation of motion, all of which manifest as painful syndromes.
Aging of the spine is characterized by two parallel but independent processes: reduction of bone mass and development of degenerative changes. These processes run contemporaneously, but at different rates. The gravity of each one is different from person to person. In addition, on an individual level, the degree of affliction is dissimilar for the various spinal levels.
The combination of degeneration and bone mass reduction contribute, to a different degree, to the development of a variety of lesions. The course these processes take as well as their biomechanical consequences are analyzed separately for each component of the spine.
INTERVERTEBRAL DISK
The intervertebral disk is composed of the gelatinous nucleus pulposus, which is surrounded by the annulus fibrosus. The nucleus contains small numbers of un-differentiated and differentiated chondrocytes and connective tissue cells. Its ground substance constitutes mostly of water, up to 88%, and a mixture of glycoproteins. There are no blood vessels or nerve endings in the nucleus. Nutrient exchange is achieved through minute pores in the end plate cartilage on either side. The hydroabsorptive properties of the nucleus’ proteins cause fluid to osmotically seep through these pores. Thus, being enclosed in a rigid case, the nucleus pulposus develops hydrostatic pressure, which contributes in resisting forces that are applied centrically (axial compression) or eccentrically (flexion-extension, lateral bending) (Fig. 1).
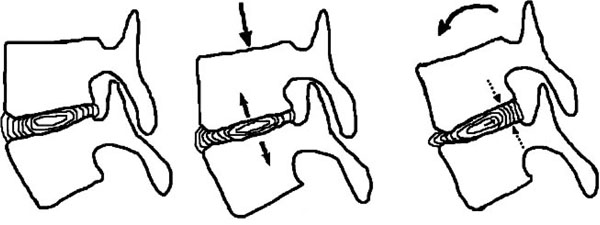
Self-regulation of the disk. Even in the steady state, the nucleus tends to expand but is restrained by the end-plates and the annulus. During axial compression, nucleus’ hydrostatic pressure rises, which counteracts the deformation. During flexion (in extension and lateral bending respectively) annulus fibers are stretched and the nucleus is pushed to the opposite side. The nucleus forces the fibers to return to their original state, thus countering the motion.
The annulus fibrosus consists of successive layers of collagen fibers. The direction of these fibers is oblique, and each layer has the opposite direction from its adjacent ones. This configuration imparts added resistance to rotation forces. Additionally, resistance to shear forces is exerted exclusively by the annulus [2], while removing the nucleus has no effect on the disk’s behaviour to these forces [3].
Disk kinematics are divided in two phases. Beginning in the neutral position, the annulus fibres are slack. The application of a load will initially cause a small deformation of the disk, up to the point where the fibres become taut. Very little resistance is exerted in that initial part of motion, as there is no elastic distension. This is the neutral zone of the disk’s range of motion, where an applied load can easily deform disk shape without significant resistance. Should the load be great enough, it will cause a greater deformation. The fibres will then stretch and the nucleus will be forced to change shape. This is the elastic zone, where the stiffness of the disk increases proportionally to the deformation caused by the applied load [1, 4].
The above mentioned facts underline that the nucleus and annulus constitute a functional unit, whose effectiveness depends on the integrity of each constituent. If the disk’s internal pressure were diminished or the integrity and structure of the annulus were disrupted, the mechanical properties of the entire disk would degrade [5].
Disk degeneration begins when the balance between synthesis and degradation of the matrix is disrupted. At a microscopic level, findings of disc degeneration include a net loss of water and glycoproteins, disruption of collagen fiber organization and increased levels of proteolytic enzymes. This alteration of disk cell’s metabolism is induced by aging and, to a large degree, depends on genetic predisposition [6]. Additionally, it has been observed that continuous or repetitive loading above or even below normal levels is related to an increase in proteolytic enzymes [7]. The concurrent degeneration of the epiphyseal plates, described in more detail later on, reduces their permeability and the consequent diminution of nutrients also has a detrimental effect [8]. Degeneration is associated with penetration of nerves and vessels in the normally avascular nucleus [9, 10]. Vascular cells produce an array of cytokines and proteases, thus stimulating further degeneration as well as inflammation. The inflammatory cells that accompany the angiogenesis in turn produce more cytokines and proteases, thus creating a vicious circle that self-amplifies. The nerve fibres that infiltrate together with the vessels carry painful stimuli, making the disk a source of pain.
These cellular and biochemical modifications bring about macroscopic anatomical alterations [6, 11]. The loss of water content causes a reduction in disk height [12]. The nucleus’ ground substance is replaced by collagen fibers and the boundary between the nucleus and the annulus becomes blurred. At the final stages of this process, the disk appears homogeneous and collapsed (black disk on MRI). The dehydrated annulus becomes more brittle, and concentric cracks and linear crevices begin to appear. Some of the changes observed on the end-plate include an increase in vascularity, thinning and formation of cracks of the cartilage, as well as sclerosis of the subchondral bone. The disk becomes gradually dislodged from the vertebral rim, and osteophytes replace Sharpey’s fibres. These degenerative changes reduce the mechanical strength of the annulus fibrosus and end-plates, both in total and locally by the formation of weak points. The result is that the disk can either bulge as a whole or protrude through said weak points. This occurs on the horizontal plane, causing either bulging or herniations, as well as on the vertical plane, causing either end plate concavity or Schmorl’s nodes.
The modification of the kinematic properties of the intervertebral disk that come about with degeneration have been thoroughly studied. Loss of height entails that the annulus fibres are more relaxed [4]. As a result a greater displacement is needed before they are stretched, and begin to resist deformation. In the early stages of degeneration therefore the neutral zone increases in all directions, like the results of experimental measurements have shown [13]. As degeneration progresses, the modulus of elasticity of the intervertebral disk increases [14], i.e. it becomes more hard and stiff, and so the range of motion begins to decline. Hence, while in the early stages of degeneration the neutral zone and accordingly instability increase, in advanced stages motion is reduced [13, 15, 16] until disk collapse and osteophyte formation result in spontaneous fusion [17].
The manner in which loads are transferred through the disk is also of particular importance. Normally, when a force is applied either centrally (axial load) or eccentrically (flexion-extension, lateral bending), it is evenly distributed on the entire disk, because of its hydrostatic properties [18]. The healthy nucleus pulposus behaves like a liquid but as degeneration progresses, it acquires the behaviour of a solid [18, 19]. Consequently, a degenerate disk no longer behaves hydrostatically [20]. Due to its low water content, application of loads causes a greater loss of height than normal, and the disk tends to bulge [12]. Since the degenerate disk can not withstand compressive loads, the surrounding structures are called to bear at least part of them. This phenomenon is called stress shielding, and it constitutes a physiological process in order to protect injured tissues [21]. Hence, while in the healthy spine only 5-10% of load is transmitted through the posterior arch, the presence of degenerate disks raises this fraction to 40% [22]. The rest of the load is not uniformly distributed, as aforementioned. The posterior part of the spinal unit receives 40% of the load, while the anterior part receives only 20% (Fig. 2) [23]. The posterior annulus fibrosus is thus more strained, which explains the increased probability of developing posterior or posterolateral herniations [24]. This unequal load distribution has an impact both in the degeneration of the posterior vertebral elements and in the morphology of osteoporotic vertebral fractures, as will be explained.
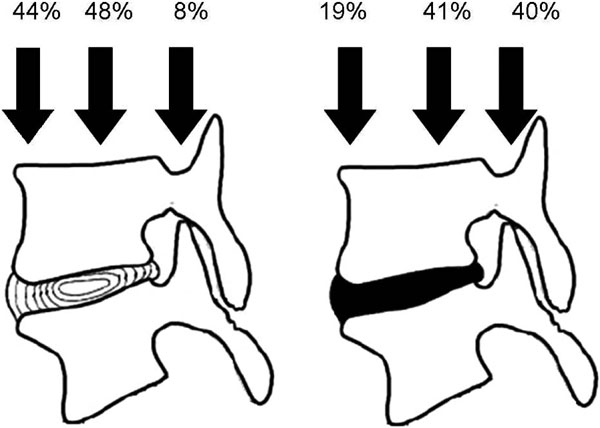
Load distribution in healthy and degenerate disks. Percentages as described in [22].
END PLATES
The end plates are composed of a thin layer of hyaline cartilage half a millimeter in thickness and a layer of cortical bone of the same thickness. They constitute strong docking surfaces for the annulus fibers. The semi-permeable cartilage allows the diffusion of water and solutes to and from the disk while it prevents loss of glycoproteins.
The distribution of subchondral bone mineralization within a joint surface is known to reflect the long term distribution of stress over an articular surface. End-plates are thinner in their central parts and thicker in the periphery [25-27]. Similarly, strength and stiffness are highest posterolaterally and lowest in the center [25, 28]. The end plates are constantly subjected to the hydrostatic pressure that develops from the nucleus [29]. It has been discovered that the thickness of the central part of the end-plate is correlated with the proteoglycan and water content of the disk [26]. Thus, in disks where hydrostatic pressure is higher, there is a corresponding increase in end-plate thickness. Additionally, it has been experimentally verified that disk degeneration, with the ensuing decline in hydrostatic pressure, causes a reduction of the end-plates’ mechanical strength, especially in the central part [30]. Even when there are no degenerative alterations, the decreasing bone density that is observed with the advancement of age causes a reduction of the end-plates’ mechanical strength [30]. The end-plate’s mechanical strength therefore depends not only on the bone mineral density of the subchondral bone but also on the remodelling that follows disk degeneration.
The degenerative changes that develop in end-plates replicate those seen in the subchondral bone of large joints [31]. Degeneration initially manifests as an increase in vascularity and subchondral bone edema. This is followed by thinning, fissuring and calcification of cartilage and ultimately subchondral bone sclerosis. These alterations are radiologically defined as Modic type changes, with type I changes representing the hypervascular, proliferative stage of degeneration and type II the subsequent degradative stage [32]. One of the deleterious effects of this process is the disruption of the permeability and hence the transport of nutrients and water to the intervertebral disk [8]. The contribution of this process in the vicious circle of disk degeneration has already been mentioned.
The main biomechanical effect that aging has on the end-plates is a reduction in their mechanical strength. Therefore, as age advances, the end-plate becomes more concave, while the disk assumes an oval shape [33]. A weak end-plate however cannot always withstand the loads to which it is subjected and may break. The results of certain experimental studies have demonstrated that the morphology of the end-plate’s mechanical failure depends mostly on the condition of the disk [34, 35]. Thus, when the disk is normal, Schmorl nodes crop up through yield points in the majority of cases. Contrarily, when the disk is degenerated, and no nucleus exists to form said nodes, central end plate fractures occur. Bone density was related with how soon these fractures occured (i.e. in how many load cycles) and if it were particularly low the entire vertebral body would break [34].
Schmorl nodes are stable lesions that remain constant over time [36]. In contrast, the occurrence of an end-plate fracture seems to lead to further degeneration of the disk [37-39]. An event that is caused or is at least promoted by bone density reduction results in more rapid development of degenerative changes. This is a prime example of how these decay processes can coexist and affect each other.
FACET JOINTS
The facet joints are true diarthrodial joints, where the joint cavity is surrounded by the joint capsule and synovial membrane, while the surfaces are covered by cartilage. Their role is to assist in load transfer, to stabilize the spinal unit in flexion and extension and to limit axial rotation [40, 41]. These facet joint features are attributed largely to their orientation in the sagittal and transverse planes. This oblique orientation contributes to the resistance to shear forces and to limitation of rotation whilst allowing a bigger range of flexion-extension [1, 40, 42].
During flexion, facet joint capsules, in addition to the posterior ligamentous structures, are stretched, thus limiting movement [43, 44]. In extension, the joint surfaces come in contact, essentially setting the limit of that motion [43, 44]. During axial rotation, initially the joint capsule on the side opposite to the rotation is stretched and begins to resist [45-47], while at the extremes of motion the inferior articular process of the rotating vertebra comes into contact with the superior process of the still vertebra beneath [41, 44]. The most important facet joint feature is the resistance they exert to shear forces. It has been ascertained experimentally that facet joints resist 77.7% of anterior and 79% of posterior shear forces [48]. In other words, they inhibit anterior translation during flexion [41] and posterior translation during extension [49]. Additionally, by inhibiting translation, they moreover avert vertebral displacement under the influence of body weight when the trunk is in a horizontal position [41].
Facet joint degeneration follows that of the disk [50-52]. As already mentioned, disk degeneration corresponds to a multiplication of the loads exerted on the facet joints. The result is the development of osteoarthritis, morphologically similar to that of the other diarthrodial joints [53, 54]. Therefore, there is denudation and ulcerative lesions of the articular cartilage, inflammatory hypertrophy of the synovial membrane, formation of osteophytes and sclerosis of the subchondral bone [54, 55]. Degeneration activates a remodelling process which on one hand stabilizes the joint but on the other causes a loss of mobility [43], in the same way as in any other joint.
The facet joints are coronally oriented in the lower lumbar vertebrae, and become more sagittaly so in higher levels [56]. This oblique orientation allows for a broader range of flexion whilst limiting rotation in the lumbar spine [1]. A number of studies have demonstrated the strong association between the increased sagittal orientation and the presence of osteoarthritis, [51, 57, 58], however it could not be verified whether this orientation predisposes to or is a consequence of osteoarthritis.
The joint capsules are richly innervated by nociceptive and autonomic nerve fibres [59-62]. They are potential sources of pain, the so called “facet joint syndrome”. Although this syndrome is controversial, the fact is that facet joint hypertrophy could cause compression of the exiting nerve root, thus resulting in lateral spinal stenosis and root pain [44]. In this particular case, facet joint hypertrophy and osteophyte formation could also lead to other clinical syndromes such as spondylolisthesis and central stenosis, which are described further on.
MUSCLES AND LIGAMENTS
Muscles and ligaments play a central role in the stability and mobility of the spinal column. Besides their mechanical action in the execution of these actions, they also constitute organs of proprioception. The nerve receptors that are responsible for proprioception and sense of motion are found in the muscles and ligaments of the spine. Activation of these receptors causes reflex responses of the muscles, either those that surround the spine or more distant ones, such as the abdominal muscles. In this way, the spine maintains its balance, performs complex movements but also protects itself from excessive or abnormal motion [21]. It is clear that degeneration of the sensory organs as well as of the end organs of these reflexes disorganizes this complex and sensitive procedure, thus disturbing both kinematics and balance. Therefore the disturbance of normal kinematics and the instability that ensue from the degeneration of these structures are not just biomechanical.
The ligaments of the spine constrain its parts and limit the range of motion in all directions. Due to their elasticity, they passively help return the spine back to the neutral position [63]. The advancement of age leads to an increased percentage of elastin to collagen, increased cross-linking of collagen fibers and finally a reduction in elasticity and strength of ligaments [64]. In addition, the reduction of intervertebral disk height concomitantly reduces the tension on the ligamentum flavum, which as a result of remodelling increases in thickness [6]. The combination of intervertebral disk height reduction together with the thickening and loss of elasticity of the ligamentum flavum result in its folding, thus contributing to the pathogenesis of spinal stenosis.
Spinal muscles are continuously active, and this muscular tone keeps the trunk upright. Without muscular support, the spine has a compression threshold of just 2 kg before buckling [65]. In both static and dynamic conditions, motion, balance and stability depend on the complex synergistic and competitive actions of the various muscle groups. With the increase in age, muscular fibers are subjected to fatty degeneration, tendons degenerate in a similar manner to ligaments and osteophytes develop on their attachment points. Consequently the generated force is reduced and additionally the motion caused by the muscles is altered, precisely because of the changes in the attachments and of the random way the fibers degenerate. The changes in biomechanics are not yet fully understood, as even the most comprehensive models of spinal muscles are until now incomplete [66].
VERTEBRAL BODY
The vertebral body receives the largest part of the load that the spine is subjected to. Size increases in the most caudal vertebrae, in agreement with the larger weight they must bear. The entire body is composed of cancellous bone, which becomes more dense and solid around the periphery, to form an outer layer. The vertebral cortex isn’t composed of cortical bone, is exceptionally thin [67], and moreover it has been discovered experimentally that it confers only 10% of the total mechanical strength of vertebrae [68].
The main factor that determines the mechanical strength of the vertebral body is its micro-architecture [69-72]. The bone trabeculae adjacent to the end plate and in the posterior part of the body are larger and their network is more dense and organized. On the contrary, the centre and anterior part of the vertebral body exhibit a smaller regional density with thinner trabeculae and a more irregular structure [73, 74]. This results in an area of reduced strength in the anterior and central part of the vertebral body. When the disk is degenerated, the architecture and the mechanical properties tend to become uniform, evening out any differences [74, 75].
The mechanical properties of the vertebral body are also directly related with its mineral density [69-72]. The relationship between bone density and compressive strength is exponential, therefore a small reduction of the former results in a big reduction of the latter [76]. With the increase in age there is an unavoidable loss of bone mass, whose rate rises steeply with the onset of menopause. Moreover, osteoporosis affects vertebral micro-architecture. The densely connected, plate-like trabeculae are transformed into discontinuous rod-like structures [77, 78]. Together with the reduction in the size and diameter of every trabecula, there is a decline in the number of horizontal trabeculae [79]. These act as cross-links in the overall structure of bone, as they resist bending of the vertical trabeculae (Fig. 3). Hence the mechanical strength decreases not only because of the smaller quantity of bone but also due to the poorer quality. The end result is that the loads that the vertebral body is subjected to in daily activities exceed its strength. Vertebral osteoporotic fractures can therefore occur even without any violence or exceptionally large loads. Since the anterior part of the vertebral body is less strong, anterior wedge fractures ought to be more frequent, as they indeed are [80].
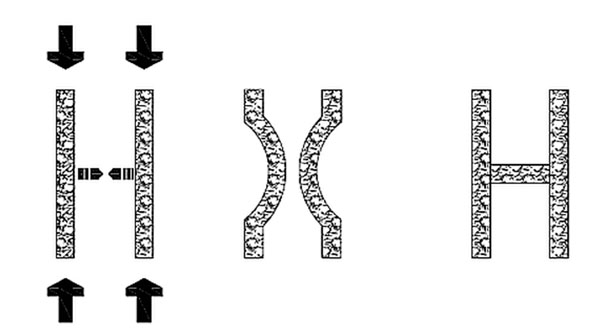
When two vertical beams are subjected to axial loading, they tend to buckle, either converging, like in the example, or diverging. A cross link prevents this deformation, thus increasing the strength of the vertical beams.
THE COMBINATION OF OSTEOPOROSIS AND DISK DEGENERATION
It is relatively uncommon for osteoporosis to be seen in patients with degenerative disk disease [81]. Some researchers have suggested that the presence of one disease excludes the existence of the other [82]. There are those who support that this finding could be a product of DEXA methodology [83]. Specifically, the antero-posterior view that is used for mineral density measurements include bony elements like the facet joints, the transverse and lateral processes and the laminae. The contribution of these elements to the vertebral structural strength is negligible, and additionally they also develop osteophytes, which falsely elevate bone mass. The subject remains controversial, although the fact remains that there are patients with both diseases.
As aforementioned, degeneration of the disk alters the way it transmits loads. The largest part of the load is distributed to the posterior part of the vertebral body, which has a higher regional bone density and greater strength. The anterior part, more fragile from the outset, now receives a smaller part of the loads, with whatever that signifies for bone remodeling [84]. Still, this redistribution of loads means that the greater portion is now exerted on the toughest part of the vertebral body [73]. Nevertheless, during flexion the anterior part receives 50-60% of loads, regardless of the state of the disk [23, 85]. In agreement with the above, biomechanical experiments have demonstrated that when the spine is axially loaded in the neutral position, compression fractures do not occur in vertebrae adjacent to degenerated disks [86]. When the spine is loaded in flexion, wedge fractures do occur, regardless of the state of the disk. Therefore, biomechanically, when both diseases co-exist, vertebrae are more stable in the neutral position but more susceptible to fracture in flexion. Just as the biomechanical effect of degenerative disk disease is ambiguous as to the overall risk of fracture, clinical studies are ambivalent as to whether disk degeneration increases [87] or reduces [88] fracture frequency.
The forces that are applied on the vertebral body and cause it to fracture are transmitted to the disk. Frank mechanical failure will occur on the vertebral body first and then on the disk, but the disk also get injured [89]. Experimental and biomechanical studies have demonstrated that a fracture has a negative effect on degenerative disk disease, as it results in changes of disk pressure, structural disorders and degeneration [37, 38, 90]. However, clinical observational studies with follow-up of up to 40 years have shown that disks adjacent to fractured vertebrae do not demonstrate degenerative changes [91, 92], except in cases where the end plate had been fractured [39]. Obviously if the disk’s structure or blood supply isn’t critically injured, the disorder of its composition or nutrient supply will not be enough to lead to degeneration.
CLINICAL SYNDROMES
Aging of the spine results in a number of painful disorders. The loss of bone mass leads to fractures of the end plate or of the vertebral body. These fractures give rise to pain, loss of height and deformity. Their impact on daily activities and quality of life is also especially detrimental.
Disk degeneration can be a source of significant pain. The infiltrating nerve fibres are activated by the metabolic by-products and inflammatory mediators found in the degenerate disk. Moreover, disk prolapse or herniation can irritate adjacent nerve roots and is in fact the leading cause of radiculopathy.
Facet degeneration is inherently a cause of pain. What’s more, facet hypertrophy can compress nerve roots, in addition to the presence of osteophytes that cause irritation. This lateral spinal stenosis gives rise to clinical symptoms similar to those of disk herniation [44].
While the disk and facet joints degenerate, they lose the ability to resist shear forces. As a result, they can no longer prevent the forward translation of the vertebra, in other words the development of degenerative spondylolisthesis. Degeneration of the ligaments that support and restrain the spinal column also contributes to this process.
Disk bulging, facet hypertrophy and ligamentum flavum hypertrophy and folding all combine to cause spinal canal stenosis. The condition is aggravated when degenerative spondylolisthesis is also present. Spinal stenosis is a serious disease, particularly when the cervical spine is affected, as it can progress to myelopathy.
The vertebral bodies in the aging spine assume a wedge-like shape, either because of micro-fractures or due to degeneration [93]. This leads to a chain distortion of the configuration of the spine. End-plate or vertebral body fractures will also cause or exacerbate this deformation. In addition, degenerative changes of posterior vertebral elements and disks in conjunction with those of spinal ligaments and muscles result in loss of balance and of normal kinematics. The end result is spinal instability, segmental or generalized. As a consequence of the combination of these alterations, the spine can be destabilized to such a degree that deformities develop such as loss of lordosis, marked kyphosis, degenerative scoliosis or combinations of the above.
The pathophysiologic and biomechanical changes that occur in the spine are the result of the increase in age and the result of other factors. These changes, often but not always, are expressed clinically as pain and disability. Nevertheless, degenerative changes or even osteoporotic fractures can be asymptomatic. The challenge a physician frequently faces in practice is to ascertain whether the patient’s symptoms are correlated with the radiologic findings or are non-specific. The diagnosis can be even more complicated when lesions are found in multiple levels. Is therefore becomes apparent that in orthopaedic practice, physical examination remains the most important and useful diagnostic tool.
CONFLICT OF INTEREST
None declared.
ACKNOWLEDGEMENT
None declared.